LIKE THE THEOREM OF THE AETHER BEFORE IT
NEWS AND VIEWS
24 APRIL 2019
Dark-matter detector observes exotic nuclear decay
A detector that was designed to probe dark matter, the ‘missing’ mass in the Universe, has seen an elusive nuclear decay called two-neutrino double electron capture — with implications for nuclear and particle physics.
Jouni Suhonen
For half a century, our view of the world has been based on the standard model of particle physics. However, this view has been challenged by theories1 that can overcome some of the limitations of the standard model. These theories allow neutrinos to be Majorana particles (that is, they are indistinguishable from their own antiparticles) and predict the existence of weakly interacting massive particles (WIMPs) as the constituents of invisible ‘dark matter’ in the Universe. Majorana neutrinos mediate a type of nuclear decay called neutrinoless double-β decay, an example of which is neutrinoless double electron capture. A crucial step towards observing this decay is to detect its standard-model equivalent: two-neutrino double electron capture. In a paper in Nature, the XENON Collaboration2 reports the first direct observation of this process in xenon-124 nuclei, using a detector that was built to detect WIMPs.
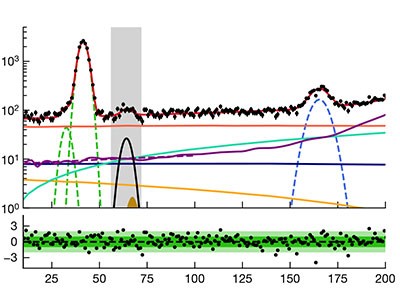
Read the paper: Observation of two-neutrino double electron capture in 124Xe with XENON1T
All known interactions in the Universe are mediated by one of four forces: electromagnetic, gravitational, strong or weak. The electromagnetic force and gravitational force, which we encounter in daily life, are long-range and can act over large distances. The strong force acts over short distances and binds together elementary particles known as quarks to form nucleons (protons and neutrons) on the femtometre scale (1 fm is 10–15 m). The weaker long-range residue of the strong force, in turn, binds nucleons into atomic nuclei. For example, this residue binds together the 124 nucleons (54 protons and 70 neutrons) of a xenon-124 nucleus. Last, the weak force is extremely short-range and causes atomic nuclei to disintegrate through a process called nuclear β-decay.
One type of β-decay is nuclear electron capture, in which a nucleus, embedded in an atom, captures an electron from the electron shells that surround it (Fig. 1a). As a result, one proton in the nucleus is converted into a neutron, and a neutrino is emitted. Electron capture, or any other form of β-decay, is known as a lowest-order weak interaction. For such processes, the decay rate of a nucleus, which is inversely proportional to the half-life of the nucleus, is proportional to the square of the weak coupling constant — a parameter that quantifies the strength of the weak force. Because this constant is small, the resulting half-life is long. For example, in the case of the electron-capture-mediated decay of iodine-124 to tellurium-124, the half-life is 4.2 days.
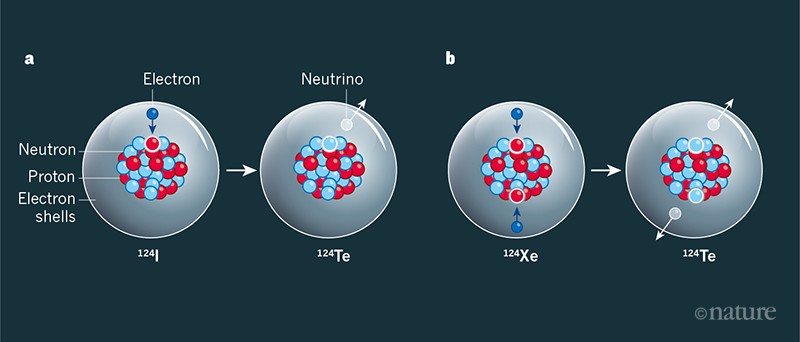
Figure 1 | Electron capture and two-neutrino double electron capture. a, An iodine-124 atom can decay with a half-life of 4.2 days to an atom of tellurium-124, through a process called electron capture. The nucleus of the iodine-124 atom captures an electron from the electron shells that surround it. A proton (circled) in the nucleus is converted into a neutron, and a neutrino is emitted. b, A xenon-124 atom cannot decay by electron capture, because of the law of energy conservation. However, it can decay with an extremely long half-life to a tellurium-124 atom, through a process known as two-neutrino double electron capture. The xenon-124 nucleus captures two electrons from the surrounding electron shells, which results in the conversion of two protons (circled) into neutrons, and the emission of two neutrinos. The XENON Collaboration2 has measured the half-life of this process to be 1.8 × 1022 years — about one trillion times the age of the Universe.
In some instances, electron capture (or any other lowest-order weak interaction) is forbidden by the law of energy conservation. Then, the nuclear decay can proceed through a weak-interaction process of the second order, for which the decay rate is proportional to the fourth power of the weak coupling constant, and the associated half-life is extremely long. An example of a second-order weak interaction is two-neutrino double electron capture, in which a nucleus captures two electrons from the electron shells that surround it, resulting in the conversion of two protons into neutrons and the emission of two neutrinos (Fig. 1b).
This process can be viewed as two simultaneous electron-capture decays that directly convert an atomic nucleus into one that has two fewer protons and two more neutrons. Each captured electron leaves a hole in the electron shell from which it came. These holes are filled by other atomic electrons, leading to the emission of X-rays and electrons called Auger electrons. Such emissions pave the way for the direct observation of two-neutrino double electron capture in a nucleus. The first experimental indications of this process were obtained for krypton-78 in direct counting experiments3,4, in which the double electron captures are registered one by one, and for barium-130 in geochemical studies5,6.
The XENON Collaboration looked for the decay of xenon-124 to tellurium-124, which occurs through two-neutrino double electron capture, using the XENON1T dark-matter detector. This instrument contains about 3 tonnes of ultra-pure liquid xenon and was designed to search for the scattering of WIMPs off xenon nuclei7. The detector is at the Gran Sasso National Laboratory, which is located under the Gran Sasso massif in central Italy, roughly 120 km from Rome. The researchers carried out a direct counting experiment in which emissions of X-rays and Auger electrons were measured to pin down the rare decay. The data were collected over one year (between 2017 and 2018) as part of the hunt for WIMPs.
Thanks to the huge amount of xenon in the detector, the authors achieved the first direct observation of two-neutrino double electron capture in xenon-124 nuclei. They measured the half-life of the process to be 1.8 × 1022 years, which is about one trillion times the age of the Universe. The successful measurement of this half-life lays the foundations for experiments that aim to detect these rare decays in other nuclei. Moreover, the researchers’ use of a WIMP-searching liquid-xenon instrument provides striking evidence of the power and versatility of such detectors. However, only four types of double-β decay can be probed by these instruments — namely, the decays of xenon-124, xenon-126, xenon-134 and xenon-136.
From the point of view of nuclear theory, the decay rates of both two-neutrino and neutrinoless double electron capture can be connected to quantities called nuclear matrix elements. Such quantities contain information about nuclear structure that is extracted from nuclear models and can be applied by researchers in the field of nuclear-structure theory. The measured two-neutrino double electron capture will help to test the various nuclear models8 that are used to calculate rates of double-β decay. Moreover, the acquired half-life data will enable model parameters to be fine-tuned, allowing scientists to more accurately predict the values of the nuclear matrix elements that are associated with neutrinoless double electron capture, as well as neutrinoless double-β decays in general. Finally, all of these factors will contribute to the accurate extraction of neutrino parameters from the data gathered by present and future neutrino experiments.
Nature 568, 462-463 (2019)
doi: 10.1038/d41586-019-01212-8
References
1.Vergados, J. D. Phys. Rep. 133, 1–216 (1986).
Article
Google Scholar
2.XENON Collaboration. Nature 568, 532–535 (2019).
Article
Google Scholar
3.Gavrilyuk, Y. M. et al. Phys. Rev. C 87, 035501 (2013).
Article
Google Scholar
4.Ratkevich, S. S. et al. Phys. Rev. C 96, 065502 (2017).
Article
Google Scholar
5.Meshik, A. P., Hohenberg, C. M., Pravdivtseva, O. V. & Kapusta, Y. S. Phys. Rev. C 64, 035205 (2001).
Article
Google Scholar
6.Pujol, M., Marty, B., Burnard, P. & Philippot, P. Geochim. Cosmochim. Acta 73, 6834–6846 (2009).
Article
Google Scholar
7.Aprile, E. et al. Phys. Rev. Lett. 121, 111302 (2018).
PubMed
Article
Google Scholar
8.Ejiri, H., Suhonen, J. & Zuber, K. Phys. Rep. 797, 1–102 (2019)
Article
Google Scholar
Download references
24 APRIL 2019
Dark-matter detector observes exotic nuclear decay
A detector that was designed to probe dark matter, the ‘missing’ mass in the Universe, has seen an elusive nuclear decay called two-neutrino double electron capture — with implications for nuclear and particle physics.
Jouni Suhonen
For half a century, our view of the world has been based on the standard model of particle physics. However, this view has been challenged by theories1 that can overcome some of the limitations of the standard model. These theories allow neutrinos to be Majorana particles (that is, they are indistinguishable from their own antiparticles) and predict the existence of weakly interacting massive particles (WIMPs) as the constituents of invisible ‘dark matter’ in the Universe. Majorana neutrinos mediate a type of nuclear decay called neutrinoless double-β decay, an example of which is neutrinoless double electron capture. A crucial step towards observing this decay is to detect its standard-model equivalent: two-neutrino double electron capture. In a paper in Nature, the XENON Collaboration2 reports the first direct observation of this process in xenon-124 nuclei, using a detector that was built to detect WIMPs.
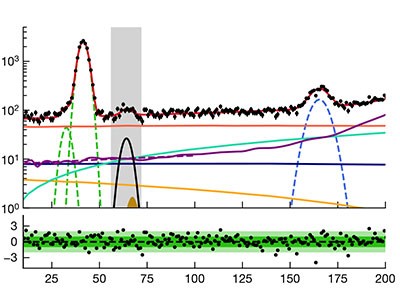
Read the paper: Observation of two-neutrino double electron capture in 124Xe with XENON1T
All known interactions in the Universe are mediated by one of four forces: electromagnetic, gravitational, strong or weak. The electromagnetic force and gravitational force, which we encounter in daily life, are long-range and can act over large distances. The strong force acts over short distances and binds together elementary particles known as quarks to form nucleons (protons and neutrons) on the femtometre scale (1 fm is 10–15 m). The weaker long-range residue of the strong force, in turn, binds nucleons into atomic nuclei. For example, this residue binds together the 124 nucleons (54 protons and 70 neutrons) of a xenon-124 nucleus. Last, the weak force is extremely short-range and causes atomic nuclei to disintegrate through a process called nuclear β-decay.
One type of β-decay is nuclear electron capture, in which a nucleus, embedded in an atom, captures an electron from the electron shells that surround it (Fig. 1a). As a result, one proton in the nucleus is converted into a neutron, and a neutrino is emitted. Electron capture, or any other form of β-decay, is known as a lowest-order weak interaction. For such processes, the decay rate of a nucleus, which is inversely proportional to the half-life of the nucleus, is proportional to the square of the weak coupling constant — a parameter that quantifies the strength of the weak force. Because this constant is small, the resulting half-life is long. For example, in the case of the electron-capture-mediated decay of iodine-124 to tellurium-124, the half-life is 4.2 days.
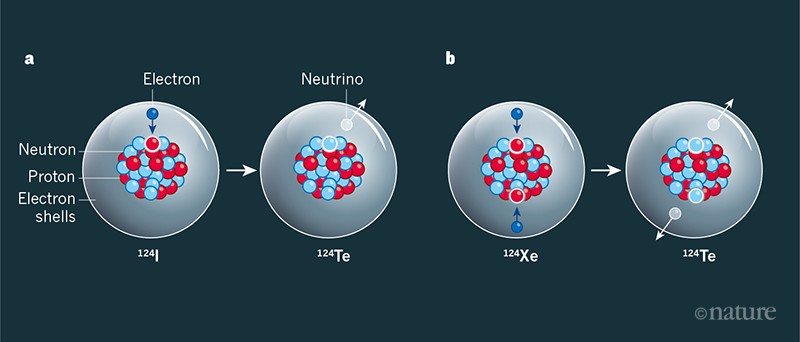
Figure 1 | Electron capture and two-neutrino double electron capture. a, An iodine-124 atom can decay with a half-life of 4.2 days to an atom of tellurium-124, through a process called electron capture. The nucleus of the iodine-124 atom captures an electron from the electron shells that surround it. A proton (circled) in the nucleus is converted into a neutron, and a neutrino is emitted. b, A xenon-124 atom cannot decay by electron capture, because of the law of energy conservation. However, it can decay with an extremely long half-life to a tellurium-124 atom, through a process known as two-neutrino double electron capture. The xenon-124 nucleus captures two electrons from the surrounding electron shells, which results in the conversion of two protons (circled) into neutrons, and the emission of two neutrinos. The XENON Collaboration2 has measured the half-life of this process to be 1.8 × 1022 years — about one trillion times the age of the Universe.
In some instances, electron capture (or any other lowest-order weak interaction) is forbidden by the law of energy conservation. Then, the nuclear decay can proceed through a weak-interaction process of the second order, for which the decay rate is proportional to the fourth power of the weak coupling constant, and the associated half-life is extremely long. An example of a second-order weak interaction is two-neutrino double electron capture, in which a nucleus captures two electrons from the electron shells that surround it, resulting in the conversion of two protons into neutrons and the emission of two neutrinos (Fig. 1b).
This process can be viewed as two simultaneous electron-capture decays that directly convert an atomic nucleus into one that has two fewer protons and two more neutrons. Each captured electron leaves a hole in the electron shell from which it came. These holes are filled by other atomic electrons, leading to the emission of X-rays and electrons called Auger electrons. Such emissions pave the way for the direct observation of two-neutrino double electron capture in a nucleus. The first experimental indications of this process were obtained for krypton-78 in direct counting experiments3,4, in which the double electron captures are registered one by one, and for barium-130 in geochemical studies5,6.
The XENON Collaboration looked for the decay of xenon-124 to tellurium-124, which occurs through two-neutrino double electron capture, using the XENON1T dark-matter detector. This instrument contains about 3 tonnes of ultra-pure liquid xenon and was designed to search for the scattering of WIMPs off xenon nuclei7. The detector is at the Gran Sasso National Laboratory, which is located under the Gran Sasso massif in central Italy, roughly 120 km from Rome. The researchers carried out a direct counting experiment in which emissions of X-rays and Auger electrons were measured to pin down the rare decay. The data were collected over one year (between 2017 and 2018) as part of the hunt for WIMPs.
Thanks to the huge amount of xenon in the detector, the authors achieved the first direct observation of two-neutrino double electron capture in xenon-124 nuclei. They measured the half-life of the process to be 1.8 × 1022 years, which is about one trillion times the age of the Universe. The successful measurement of this half-life lays the foundations for experiments that aim to detect these rare decays in other nuclei. Moreover, the researchers’ use of a WIMP-searching liquid-xenon instrument provides striking evidence of the power and versatility of such detectors. However, only four types of double-β decay can be probed by these instruments — namely, the decays of xenon-124, xenon-126, xenon-134 and xenon-136.
From the point of view of nuclear theory, the decay rates of both two-neutrino and neutrinoless double electron capture can be connected to quantities called nuclear matrix elements. Such quantities contain information about nuclear structure that is extracted from nuclear models and can be applied by researchers in the field of nuclear-structure theory. The measured two-neutrino double electron capture will help to test the various nuclear models8 that are used to calculate rates of double-β decay. Moreover, the acquired half-life data will enable model parameters to be fine-tuned, allowing scientists to more accurately predict the values of the nuclear matrix elements that are associated with neutrinoless double electron capture, as well as neutrinoless double-β decays in general. Finally, all of these factors will contribute to the accurate extraction of neutrino parameters from the data gathered by present and future neutrino experiments.
Nature 568, 462-463 (2019)
doi: 10.1038/d41586-019-01212-8
References
1.Vergados, J. D. Phys. Rep. 133, 1–216 (1986).
Article
Google Scholar
2.XENON Collaboration. Nature 568, 532–535 (2019).
Article
Google Scholar
3.Gavrilyuk, Y. M. et al. Phys. Rev. C 87, 035501 (2013).
Article
Google Scholar
4.Ratkevich, S. S. et al. Phys. Rev. C 96, 065502 (2017).
Article
Google Scholar
5.Meshik, A. P., Hohenberg, C. M., Pravdivtseva, O. V. & Kapusta, Y. S. Phys. Rev. C 64, 035205 (2001).
Article
Google Scholar
6.Pujol, M., Marty, B., Burnard, P. & Philippot, P. Geochim. Cosmochim. Acta 73, 6834–6846 (2009).
Article
Google Scholar
7.Aprile, E. et al. Phys. Rev. Lett. 121, 111302 (2018).
PubMed
Article
Google Scholar
8.Ejiri, H., Suhonen, J. & Zuber, K. Phys. Rep. 797, 1–102 (2019)
Article
Google Scholar
Download references