In which the author travels to South Dakota to visit a gold mine—housing LUX.
MATTHEW FRANCIS - 9/6/2021, 6:39 AM
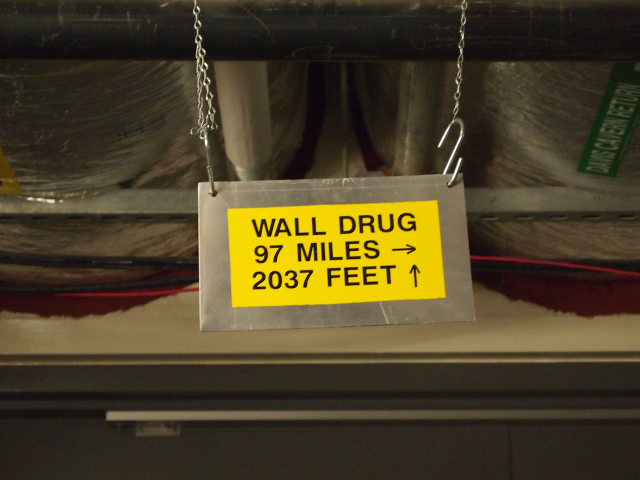
A mile below ground, a sign hangs over the door to the LUX dark matter experiment telling visitors how far to Wall Drug—in both dimensions.
Matthew R. Francis
Update, Sept. 6, 2021: It's Labor Day Weekend in the US, and even though most of us are continuing to call home "the office," Ars staff is taking a long weekend to rest and relax. And given we can't travel like we could during Labor Day Weekends past, we thought we'd revisit one of our favorite trips from the archives. This story on our adventure to the Large Underground Xenon (LUX) dark matter experiment in South Dakota originally ran in July 2014, and it appears unchanged below.
One of the quietest, darkest places in the cosmos isn’t out in the depths of space. It’s at the center of a tank of cold liquid xenon in a gold mine deep under the Black Hills of South Dakota. It needs to be that quiet: any stray particles could confuse the detectors lining the outside of the tank. Those detectors are looking for faint, rare signals, ones that could reveal the presence of dark matter.
The whole assembly—the container of liquid and gaseous xenon, the water tank enveloping that, and all the detectors—is called the Large Underground Xenon (LUX) dark matter experiment. So far, LUX hasn’t found anything, but the days of its operation are just beginning: the detector was installed and started operations just last year.
Though still relatively young, LUX has already set many standards for hunting for dark matter particles. When I visited, the facility was gearing up for the next data collection run, one that will involve 300 days of constant operation. The size and sensitivity of the experiment, its designers’ dedication to understanding any noise sources, and the relative simplicity of the detector lead many to hope that if there’s any dark matter to be found, LUX—or its successor—will find it.
(I’ll use “detector” to describe LUX as a whole in addition to the individual photon detectors that are the business-end of the experiment. I hope the context will make it clear which is which.)
That last "if" is a big one, of course. Dark matter is remarkable for its invisibility—it neither absorbs nor emits light of any wavelength. We know about it through its gravitational action, the way it shaped galaxies, organized the largest objects in the Universe, and affected the spectrum of light from the early days of the cosmos. Based on the structure of galaxies, astronomers suspect it is made of particles. But how massive those particles are, how many types might exist, and how they interact are still matters of some conjecture.
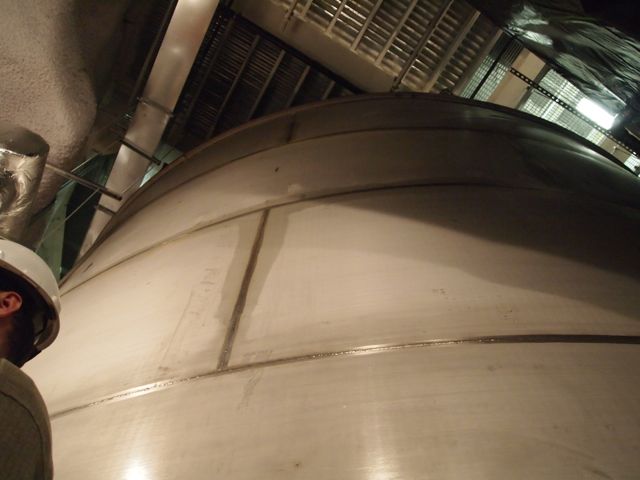
The LUX experiment is inside a metal tank containing 71,600 gallons of pure water, so the xenon detector isn't visible. The head at lower left is LUX researcher Rick Gaitskell.
Matthew R. FrancisIf, for example, dark matter doesn’t interact with ordinary matter on any scale our experimental cleverness can currently reach, LUX will turn up nothing, no matter how sophisticated the experiment is.
Advertisement
Rick Gaitskell, one of the principle researchers on LUX, is a professor at Brown University in the United States. (He was born and educated in England, a heritage that came through in his sartorial choice of green flannel three-piece suit with bright red socks.) He told Ars, “I’ve been looking for dark matter for 27 years. We’ve had a number of results over the years where we’ve either ended up seeing nothing or one or two cases we’ve seen something that looked actually rather exciting, in that it showed initially many of the features you might associate with a dark matter interaction.” However, none of those have been confirmed by later experiments—and most now have mundane explanations.
This past frustration is part of what drives Gaitskell. He quit another experiment when he concluded it was likely never to succeed: the detection rate, based on calculations, would likely be less than one dark matter particle per century for each kilogram of detector material. For most detector materials, that implies a prohibitively high cost. If you want to find dark matter on the scale of a year or two, you’d need a detector with masses of several metric tons. But mass is where LUX and its siblings—such as the XENON100 experiment at Gran Sasso in Italy—excel. Using xenon as the detection medium has many advantages, including the ability to build large.
Unicorns, Wall Drug, and dark matter
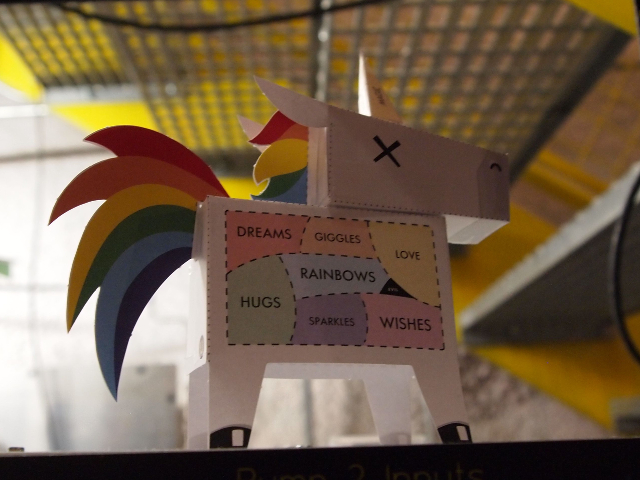
The lab housing LUX has many small paper unicorns sitting on top of server racks and other equipment.
Matthew R. FrancisTo understand how LUX hunts dark matter, I decided to visit the lab and see for myself. It's not exactly in a location that makes you think of physics.
I arrived in South Dakota during the dying throes of winter; at the end of March, the Black Hills were still snow-covered, but the warmer air carried tantalizing hints of spring. Thankfully, the flights fell between late-season snowfalls, though it snowed enough to cover my rental car during my day underground at LUX. The Black Hills are mountains in miniature, steep rocky peaks topping out lower than the foothills of the grander front range of the Rockies or the Tetons. Nevertheless, they are mountains with all that entails: sudden snowstorms, gates that can close off the roads in the case of severe weather, and “falling rocks” signs everywhere.
LUX is part of the Sanford Underground Research Facility (SURF), housed at the now-defunct Homestake gold mine in the town of Lead. (The irony of a gold mine in Lead vanished quickly. The name is pronounced “leed," not like the metal.) The whole region cashes in on its Wild West past, especially the adjacent town of Deadwood. Every hotel and gas station has slot machines, and innumerable billboards advertise big payouts at the casinos. South Dakota in general has unrestrictive laws about billboards, as anyone crossing the state by car knows. There are signs for Wall Drug, Mount Rushmore, and various other tourist destinations that nearly dwarf the attractions themselves. Continuing this trend, the lab has its own Wall Drug sign, showing the distance to be 97 miles away horizontally, plus 2037 feet vertically. Advertisement
By advertising standards, Sanford and LUX are relatively low key. The lab generally isn’t open to the public. To get to it, I had to drive through a residential neighborhood up steep (and at that time of year) snow-packed streets. Because access to the underground portion of the lab requires running the mining elevators—known as “cages”—I had to report at 6am for safety training. Even with jet-lag more or less in my favor, that was still rather early to be functional, though I had it better than the BBC film crew that flew in from the UK.
The safety briefing consisted largely of a video letting us know what we should do if we needed to evacuate the lab. We signed a waiver. We even had to affirm that we would make “intelligent choices,” a seemingly futile thing to ask a bunch of journalists with no mining experience among them. Finally, before descending, we had to don rubber steel-toed boots, coveralls, safety goggles, hard hats with lanterns, and a special emergency breathing apparatus clipped to a heavy utility belt. We made for a sexy-looking crew by the end of the process.
The cages themselves are large enough for about 15 people, with tracks in the floor for the mining carts. They have no built-in lights, so during the 10-minute descent, many of the crew turned on their hard-hat lanterns. (This is not a trip for those with claustrophobia.) Even the motors raising and lowering the cages are vintage: they were built in 1939, and the cable spools are cast iron. The gauges showing the position of the elevators are huge disks with pointers, another delightfully analog touch.
Gaitskell brought an adapted airplane altimeter into our cage to show in real time how far we were descending. Since the top of the mine is roughly a mile above sea-level and the lab is about a mile below ground, we nearly reached the elevation of my home city of Richmond, Virginia. My ears certainly registered the change in air pressure.
However rustic the approach, the lab itself is a typical, sleek modern facility: all shiny pipes, metal stairways, and tile floors. The typical accoutrements of office life abound. There are computers, white boards, water coolers, and (that most necessary piece of lab equipment) espresso machines all underground. University College London PhD student Sally Shaw told me “You kind of forget you’re underground down there.” Additionally, the researchers have adorned the lab with personal touches. A warning sign admonishes visitors to not feed the scientists, and when I looked around, I spotted a few paper unicorns sitting on various shelves. Shaw said the unicorns probably started as a late-night boredom project, but they grew into an inside joke. After all, hunting for dark matter is like looking for unicorns.
Be vewwy vewwy quiet, we're hunting WIMPs
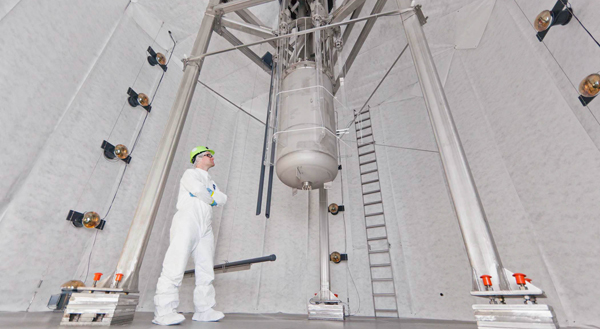
The LUX detector.
Matt Kapust/Sanford Underground Research FacilityLUX is designed to hunt for weakly interacting massive particles, or WIMPs (one of the more unfortunate acronyms in cosmology). As the name suggests, WIMPs should interact with ordinary matter, albeit infrequently and weakly—what physicists call relatively low energy scales. From astronomical surveys, researchers also predict they should have a relatively large mass and move slowly compared to the speed of light. (We’ll discuss some alternative ideas in the third installment of this series.)
Beyond those basic criteria, however, LUX is designed to be as particle-agnostic as possible. Any particle that fits the basic description should register in the detector.
That could be critical, as "WIMP" is a fairly generic term that encompasses a number of hypothetical particle types predicted by theory. For example, the category includes particles predicted in some versions of supersymmetry; the “lightest supersymmetric partner” is sometimes listed as the most likely candidate for WIMP dark matter. But this isn't the only option that's been suggested.
The general properties of WIMPs don’t come from a particular theory, but from a general idea of how they should behave. WIMP models involve particles ranging from about 40 to 1,000 times the mass of a proton, though lower-mass versions are also possible—an important consideration. No matter what, though, experiments are looking for particles moving relatively slowly compared to the speed of light, meaning they will deposit very little energy into the detector.
While LUX consists of 368 kilograms of xenon, not all of the mass is used for dark matter hunting. In fact, most of the volume of the experiment consists of shielding. Each layer blocks more particles that could interfere with WIMP searches. Jim Dobson, a postdoctoral researcher at the University of Edinburgh and University College London, explained the process. “We go underground to get away from the majority of the cosmic rays. Then you build a huge water tank to mitigate the remaining cosmics that get through and any kind of radioactive materials in the cavern.” The biggest annoyances are gamma rays and neutrons, which are rare that far below ground but problematic. (Neutrinos, which like WIMPs are electrically neutral, are too high energy to be confused with dark matter.)
Finally, the xenon is part liquid, part gas. The gas portion is the final barrier. “We only look for dark matter in the inner region of the detector, where the xenon itself acts as … the most effective final shield towards radiation. When you get right in the center, you have almost zero background in that region that could fake a WIMP experiment.” This is a key difference with some other experiments, which showed something that looked like a WIMP, but it was actually the result of a more mundane process in the detector itself. LUX is designed to sidestep those problems.
Most WIMPs entering LUX won’t hit anything, but given enough time, we might get lucky. Xenon is very nonreactive. It is an inert gas, meaning that it doesn’t form chemical bonds easily. If a WIMP strikes the nucleus of a xenon atom, it transfers some energy. This results in a number of events—the rebound of the nucleus, the loss of one electron, and the production of some photons. Fortunately, we're very good at detecting photons and stray electrons.
However, as Dobson said, “The challenge of that is reading out the signal from the middle of the detector. How do you see a few photons of light right in the middle, how do you see a few electrons being created by that interaction? This is why you have to purify the xenon constantly." And that’s part of graduate student Sally Shaw’s job: monitoring the purification process. While she laughingly downplays the importance of her efforts—“get the PhD student to do the monkey work”—it’s obviously something that needs doing. When all goes well, “you could see the concentration of the nitrogen dropping each time we took a sample, see [the xenon] getting purer.” Advertisement
Pure xenon is essential because it is remarkably transparent to both the light and electrons ejected from a possible WIMP collision. Anything else, particularly nitrogen (which can be outgassed from the tank walls), clouds the chamber, and the tiny signal could disappear—a major tragedy in experimental terms. Pure xenon also will not absorb stray electrons; there’s no place for the atom to hold them. Applying a very small electric field across the tank gently encourages those electrons to move upward until they can be registered at the detector at LUX’s top surface.
The challenge in LUX, then, is to pick up that single electron and figure out where it came from inside the tank of liquid xenon. That in turn allows researchers to measure how much energy the WIMP transferred to the xenon atom and work backward to figure out how much mass it has. The mass, of course, is one of the important parameters characterizing any particle, setting the scale for how much energy was required to create it in the Universe’s first instants of existence.
So underground, even hipsters don't know about it
Beyond the detector, LUX is a challenging project with many unusual aspects. While engineering is always an aspect of big physics experiments, any facility deep underground will have more than its share of complications. The Sanford lab occupies about 12 miles of the original tunnels from the Homestake gold mine. While that’s a tiny fraction of the total mine, maintaining a livable working environment requires keeping the air circulating and breathable, pumping water out (anything that deep underground will flood if left alone), as well as providing electricity and communications.
When you consider workers are present in the mine for long shifts, something has to be done with their bodily wastes. Unlike more ordinary trash, it’s not something you can carry back to the surface in a receptacle. (In the brief periods I was in the lab, I didn’t need to use the Port-a-potties or combustion toilets, though perhaps I should have taken care of business in the name of research—you, dear readers, deserve no less.)
Rick Gaitskell told Ars, “When you start as a physicist, you sort of assume you’ll spend your time on very esoteric calculations. The reality is I know more about pumping water, mining operations, how to treat water—which is fascinating—when you’re pulling over half a million gallons a day out of the mine, just the business of treating [it] is fascinating technology.” For comparison, that’s roughly the same as pumping and chemically treating enough water to fill an Olympic-sized swimming pool.
Safety is an overwhelming concern as well. The first morning of my visit, we were delayed in going below ground for several hours after a fiber-optic cable used for monitoring carbon monoxide levels was severed. While nobody I spoke to thought that meant conditions were dangerous in the mine, every piece of protocol is designed to make sure people are safe.
While the modern incarnation of the lab is very recent—LUX wasn’t installed underground until winter of 2013, and the other experiments are still under construction—Homestake has a storied history as a physics facility. Ray Davis constructed a neutrino detector in the mine back in the late 1960s; the results he obtained led him and collaborator John Bahcall to recognize that two-thirds of the expected neutrinos from the Sun were absent. That discovery led eventually to our modern understanding of neutrino oscillation and a Nobel Prize for Davis in 2002. (Bahcall was unfairly left out of the award.) LUX is housed in the same artificial cave blasted out of the rock for Davis’ experiment; today that part of the mine is known as the Davis Campus in his honor.
Gaitskell said that Homestake ceased mining operations in 2002 when gold prices tanked and looked like they would remain low indefinitely, but it took several more years for the Sanford lab to negotiate access. During that time, water rose to fill much of the mine, drowning the tunnels until the Davis Campus was about 400 feet below the surface. The pumps needed to be restarted and the area cleaned before any physics experiments could become more than a dream. Advertisement
Beyond its extreme location, another difference between LUX and other particle physics experiments is the way responsibility is doled out. Both Dobson and Shaw commented on how quickly junior researchers were allowed to take responsibility for important aspects of the experiment. Shaw in particular is a first-year PhD student. In most labs, she wouldn’t be participating in on-site research until later in her program, but at LUX that’s more standard. She told Ars, “It was a bit of a shock that I could come out so quickly—only a few months in, and they said straight away, ‘oh here’s some responsibility’—woo! I think it’s good, it was a real shock that the people I had heard on telephone conferences I thought were postdocs or quite old turned out to just be PhD students because they’re really pulling their weight.”
Dobson concurred. “By the time they’re at the end of their PhDs, [the students] really own whole subsystems. I was really surprised when I joined; it’s really cool how much responsibility they’re given. Along with the [senior researchers], the mature PhD students are the ones who know the most.” That’s in contrast to many of the big particle experiments such as the detectors at CERN, where hundreds of researchers may be involved, and there’s little room for junior students to contribute anything other than basic grunt-work.
First results and the future
In its preliminary run in 2013, LUX took data for about 90 days. Already during that relatively short time of operation, the detector pushed the limits of what other experiments had done, both in terms of the energy range of searching and the control of potential false signals. Again, the extremely quiet center of the xenon tank is LUX’s advantage. Any signal the researchers might see would be hard to mistake for anything other than a WIMP. The fact that they didn’t see anything during those 90 days isn’t a mark of failure—it's a sign everything is working as expected.
But of course, the LUX team wants to see dark matter, that’s why the upcoming 300-day run is crucial. LUX will need to run reliably for 24 hours a day for the better part of a year, which will leave us with a lot of clean data for researchers to work with. If any WIMP signals are present, 300 days should be sufficiently long to tell us something about what kind of particle is producing them. If nothing is there, on the other hand… it’s going to rule out some possibilities. Null experiments can be as valuable to science as those that discover something new.
And LUX isn’t the end of the road. Gaitskell told Ars, “Even at the time we were putting LUX together, we knew there would be a successor experiment. The reason is that if you see anything in LUX, you want to make detailed measurements of it. If you don’t see anything, you want to increase your sensitivity.” If LUX does detect dark matter during its 300-day run, the upgraded experiment—known as LZ, for LUX-ZEPLIN—will be even better able to characterize it. (ZEPLIN was a similar xenon-based dark matter detector in northwest England; LZ will combine resources from both existing projects.) The goal of LZ will be to help us understand how the particles interact, how much mass they have, what kind of spin they possess… all the characteristics of the particles to help fit them into what we know about other matter.
If all goes as planned, LZ will also occupy the Davis Campus where LUX currently resides. The detector will consist of at least five metric tons of xenon, more than a 10-fold increase in volume over LUX, with a corresponding increase in sensitivity. As Jim Dobson said, with LZ “you’re getting close to seeing coherent neutrino scattering from atmospheric neutrinos.” That’s nearly the practical limit of anything you could do with a dark matter experiment. If the researchers don’t see anything with LUX and LZ, there’s probably nothing to be seen in that energy range. But Dobson is sanguine. “If you discover something and the signal is big enough, you can start actually doing a lot more physics and exploring what that signal means. Exciting times!”
Further reading: Physical Review Letters, 2014. DOI: (About DOIs).
No comments:
Post a Comment