New superconducting magnet breaks magnetic field strength records, paving the way for practical, commercial, carbon-free power.
Watch Video
David Chandler | MIT News Office
Publication Date:September 8, 2021
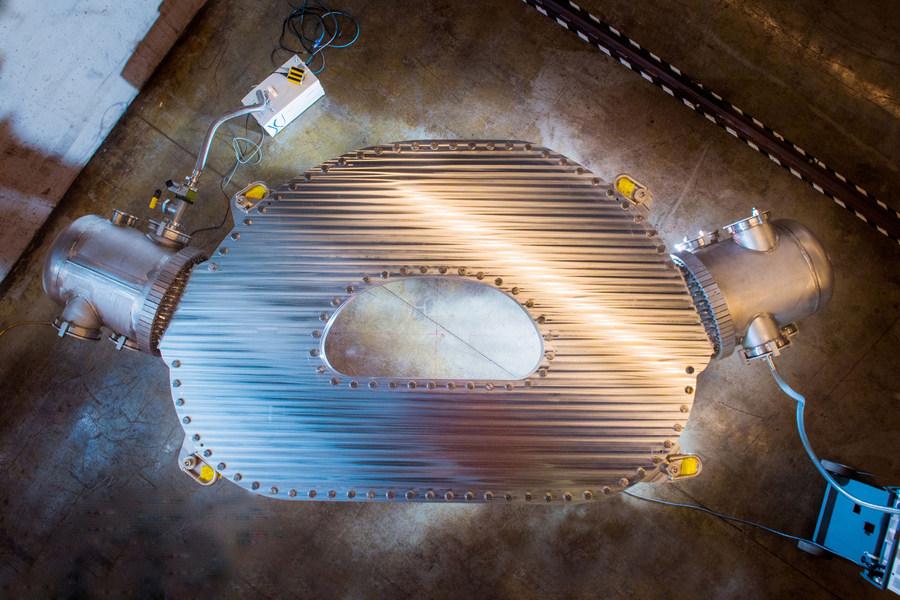
Caption:This large-bore, full-scale high-temperature superconducting magnet designed and built by Commonwealth Fusion Systems and MIT’s Plasma Science and Fusion Center (PSFC) has demonstrated a record-breaking 20 tesla magnetic field. It is the strongest fusion magnet in the world.
Credits:Credit: Gretchen Ertl, CFS/MIT-PSFC, 2021
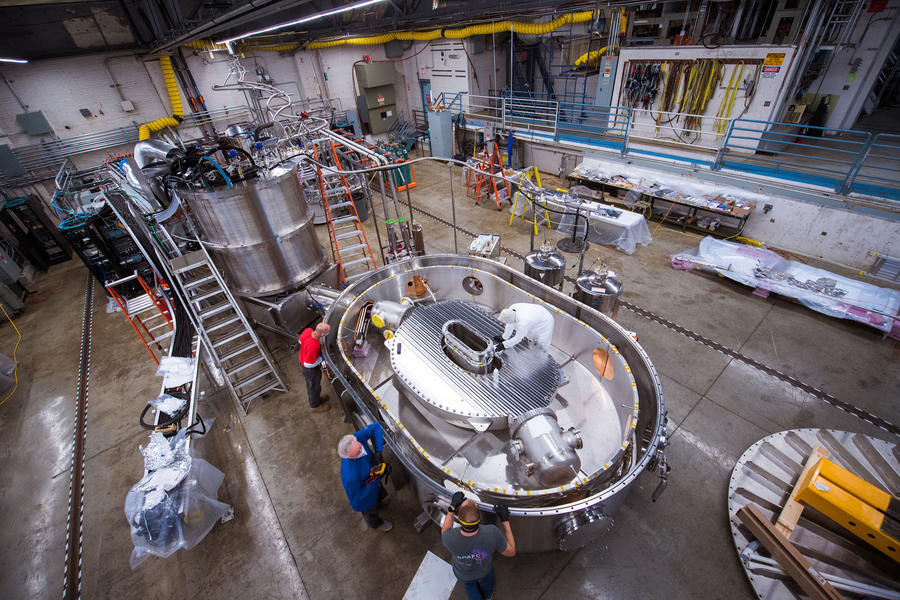
Caption:Collaborative team working on the magnet inside the test stand housed at MIT. Research, construction and testing of this magnet has been the single largest activity for the SPARC team, which has grown to include 270 members.
Credits:Credit: Gretchen Ertl, CFS/MIT-PSFC, 2021

Caption:Spool of high-temperature superconducting tape used in the new class of fusion magnet. The magnet built and tested by CFS and MIT contains 267 km (166 mi) of tape, which is the distance from Boston, MA to Albany, NY.
Credits:Credit: Gretchen Ertl, CFS/MIT-PSFC, 2021

Caption:A team of engineers and scientists from CFS and MIT’s PSFC lower the superconducting magnet into the test stand in which the magnet was cooled and powered to produce a magnetic field of 20 tesla.
Credits:Credit: Gretchen Ertl, CFS/MIT-PSFC, 2021

Caption:Director of the PSFC Dennis Whyte (L) and CEO of CFS Bob Mumgaard (R) in the test hall at MIT’s Plasma Science and Fusion Center. The collaboration which began over three years ago with the formation of Commonwealth Fusion Systems now moves to the next phase, building SPARC, which will be the world’s device to create and confine a plasma that produces net fusion energy.
Credits:Credit: Gretchen Ertl, CFS/MIT-PSFC, 2021

Caption:Rendering of SPARC, a compact, high-field, tokamak, currently under design by a team from the Massachusetts Institute of Technology and Commonwealth Fusion Systems. Its mission is to create and confine a plasma that produces net fusion energy.
Credits:Credit: T. Henderson, CFS/MIT-PSFC, 2020
It was a moment three years in the making, based on intensive research and design work: On Sept. 5, for the first time, a large high-temperature superconducting electromagnet was ramped up to a field strength of 20 tesla, the most powerful magnetic field of its kind ever created on Earth. That successful demonstration helps resolve the greatest uncertainty in the quest to build the world’s first fusion power plant that can produce more power than it consumes, according to the project’s leaders at MIT and startup company Commonwealth Fusion Systems (CFS).
That advance paves the way, they say, for the long-sought creation of practical, inexpensive, carbon-free power plants that could make a major contribution to limiting the effects of global climate change
“Fusion in a lot of ways is the ultimate clean energy source,” says Maria Zuber, MIT’s vice president for research and E. A. Griswold Professor of Geophysics. “The amount of power that is available is really game-changing.” The fuel used to create fusion energy comes from water, and “the Earth is full of water — it’s a nearly unlimited resource. We just have to figure out how to utilize it.”
Developing the new magnet is seen as the greatest technological hurdle to making that happen; its successful operation now opens the door to demonstrating fusion in a lab on Earth, which has been pursued for decades with limited progress. With the magnet technology now successfully demonstrated, the MIT-CFS collaboration is on track to build the world’s first fusion device that can create and confine a plasma that produces more energy than it consumes. That demonstration device, called SPARC, is targeted for completion in 2025
“The challenges of making fusion happen are both technical and scientific,” says Dennis Whyte, director of MIT’s Plasma Science and Fusion Center, which is working with CFS to develop SPARC. But once the technology is proven, he says, “it’s an inexhaustible, carbon-free source of energy that you can deploy anywhere and at any time. It’s really a fundamentally new energy source.”
Whyte, who is the Hitachi America Professor of Engineering, says this week’s demonstration represents a major milestone, addressing the biggest questions remaining about the feasibility of the SPARC design. “It’s really a watershed moment, I believe, in fusion science and technology,” he says.
On Sept. 5, 2021, for the first time, a large high-temperature superconducting electromagnet was ramped up to a field strength of 20 tesla, the most powerful magnetic field of its kind ever created on Earth. That successful demonstration helps resolve the greatest uncertainty in the quest to build the world’s first fusion power plant that can produce more power than it consumes, according to the project’s leaders at MIT and startup company Commonwealth Fusion Systems.
The sun in a bottle
Fusion is the process that powers the sun: the merger of two small atoms to make a larger one, releasing prodigious amounts of energy. But the process requires temperatures far beyond what any solid material could withstand. To capture the sun’s power source here on Earth, what’s needed is a way of capturing and containing something that hot — 100,000,000 degrees or more — by suspending it in a way that prevents it from coming into contact with anything solid.
That’s done through intense magnetic fields, which form a kind of invisible bottle to contain the hot swirling soup of protons and electrons, called a plasma. Because the particles have an electric charge, they are strongly controlled by the magnetic fields, and the most widely used configuration for containing them is a donut-shaped device called a tokamak. Most of these devices have produced their magnetic fields using conventional electromagnets made of copper, but the latest and largest version under construction in France, called ITER, uses what are known as low-temperature superconductors.
The major innovation in the MIT-CFS fusion design is the use of high-temperature superconductors, which enable a much stronger magnetic field in a smaller space. This design was made possible by a new kind of superconducting material that became commercially available a few years ago. The idea initially arose as a class project in a nuclear engineering class taught by Whyte. The idea seemed so promising that it continued to be developed over the next few iterations of that class, leading to the ARC power plant design concept in early 2015. SPARC, designed to be about half the size of ARC, is a testbed to prove the concept before construction of the full-size, power-producing plant.
Until now, the only way to achieve the colossally powerful magnetic fields needed to create a magnetic “bottle” capable of containing plasma heated up to hundreds of millions of degrees was to make them larger and larger. But the new high-temperature superconductor material, made in the form of a flat, ribbon-like tape, makes it possible to achieve a higher magnetic field in a smaller device, equaling the performance that would be achieved in an apparatus 40 times larger in volume using conventional low-temperature superconducting magnets. That leap in power versus size is the key element in ARC’s revolutionary design.
The use of the new high-temperature superconducting magnets makes it possible to apply decades of experimental knowledge gained from the operation of tokamak experiments, including MIT’s own Alcator series. The new approach, led by Zach Hartwig, the MIT principal investigator and the Robert N. Noyce Career Development Assistant Professor of Nuclear Science and Engineering, uses a well-known design but scales everything down to about half the linear size and still achieves the same operational conditions because of the higher magnetic field.
A series of scientific papers published last year outlined the physical basis and, by simulation, confirmed the viability of the new fusion device. The papers showed that, if the magnets worked as expected, the whole fusion system should indeed produce net power output, for the first time in decades of fusion research.
Martin Greenwald, deputy director and senior research scientist at the PSFC, says unlike some other designs for fusion experiments, “the niche that we were filling was to use conventional plasma physics, and conventional tokamak designs and engineering, but bring to it this new magnet technology. So, we weren’t requiring innovation in a half-dozen different areas. We would just innovate on the magnet, and then apply the knowledge base of what’s been learned over the last decades.”
That combination of scientifically established design principles and game-changing magnetic field strength is what makes it possible to achieve a plant that could be economically viable and developed on a fast track. “It’s a big moment,” says Bob Mumgaard, CEO of CFS. “We now have a platform that is both scientifically very well-advanced, because of the decades of research on these machines, and also commercially very interesting. What it does is allow us to build devices faster, smaller, and at less cost,” he says of the successful magnet demonstration.
Proof of the concept
Bringing that new magnet concept to reality required three years of intensive work on design, establishing supply chains, and working out manufacturing methods for magnets that may eventually need to be produced by the thousands.
“We built a first-of-a-kind, superconducting magnet. It required a lot of work to create unique manufacturing processes and equipment. As a result, we are now well-prepared to ramp-up for SPARC production,” says Joy Dunn, head of operations at CFS. “We started with a physics model and a CAD design, and worked through lots of development and prototypes to turn a design on paper into this actual physical magnet.” That entailed building manufacturing capabilities and testing facilities, including an iterative process with multiple suppliers of the superconducting tape, to help them reach the ability to produce material that met the needed specifications — and for which CFS is now overwhelmingly the world’s biggest user.
They worked with two possible magnet designs in parallel, both of which ended up meeting the design requirements, she says. “It really came down to which one would revolutionize the way that we make superconducting magnets, and which one was easier to build.” The design they adopted clearly stood out in that regard, she says.
In this test, the new magnet was gradually powered up in a series of steps until reaching the goal of a 20 tesla magnetic field — the highest field strength ever for a high-temperature superconducting fusion magnet. The magnet is composed of 16 plates stacked together, each one of which by itself would be the most powerful high-temperature superconducting magnet in the world.
“Three years ago we announced a plan,” says Mumgaard, “to build a 20-tesla magnet, which is what we will need for future fusion machines.” That goal has now been achieved, right on schedule, even with the pandemic, he says.
Citing the series of physics papers published last year, Brandon Sorbom, the chief science officer at CFS, says “basically the papers conclude that if we build the magnet, all of the physics will work in SPARC. So, this demonstration answers the question: Can they build the magnet? It’s a very exciting time! It’s a huge milestone.”
The next step will be building SPARC, a smaller-scale version of the planned ARC power plant. The successful operation of SPARC will demonstrate that a full-scale commercial fusion power plant is practical, clearing the way for rapid design and construction of that pioneering device can then proceed full speed.
Zuber says that “I now am genuinely optimistic that SPARC can achieve net positive energy, based on the demonstrated performance of the magnets. The next step is to scale up, to build an actual power plant. There are still many challenges ahead, not the least of which is developing a design that allows for reliable, sustained operation. And realizing that the goal here is commercialization, another major challenge will be economic. How do you design these power plants so it will be cost effective to build and deploy them?”
Someday in a hoped-for future, when there may be thousands of fusion plants powering clean electric grids around the world, Zuber says, “I think we’re going to look back and think about how we got there, and I think the demonstration of the magnet technology, for me, is the time when I believed that, wow, we can really do this.”
The successful creation of a power-producing fusion device would be a tremendous scientific achievement, Zuber notes. But that’s not the main point. “None of us are trying to win trophies at this point. We’re trying to keep the planet livable.”
Is The World Investing Enough In Nuclear Fusion Research?
The old cliched joke in newsrooms used to be: “Nuclear fusion is 30 years away...and always will be.” While that cheeky adage has continued to ring true even as scientists made small advancements and mini-breakthroughs in over the last 100 years, things are finally starting to catch traction in the highly experimental world of man-made nuclear fusion.
A recent record-breaking experiment at the United States National Ignition Facility (NIF), based at Lawrence Livermore National Laboratory in California got remarkably close to achieving net energy creation from their laser-based fusion experiment. The flash of light and energy which ensued lasted only a tiny fraction of a second, but which triggered a self-sustaining chain of nuclear fusion reactions -- the holy grail of nuclear fusion exploits. That reaction, which showed a 1,000% increase in energy release since 2011 experiments, came incredibly close to proving that net energy gain through fusion is possible in a lab setting.
“The pace of improvement in energy output has been rapid, suggesting we may soon reach more energy milestones, such as exceeding the energy input from the lasers used to kickstart the process,” Professor Jeremy Chittenden, co-director of the Centre for Inertial Fusion Studies at Imperial College London, was quoted last month by phys.org.
It’s nearly impossible to overstate the potential disruption that achieving net energy gain and, eventually, commercial nuclear fusion would have for the global energy landscape, the worldwide economy, and climate change. It would change everything. Unlike nuclear fission, which currently powers nuclear power plants around the world, nuclear fusion is a completely clean form of energy production, leaving no hazardous or radioactive waste behind. Its hydrogen-based fuel sources, deuterium and tritium, are plentiful and will be in abundance for thousands of years. And, unlike wind and solar, nuclear fusion requires relatively little land to operate.
While nuclear fission entails capturing the energy released by splitting unstable atoms, nuclear fusion is the process of combining two smaller atoms into one larger one, a process which releases several times more energy. This is the process that powers our own sun and stars and which created most of the atoms that you and I are made of. Nuclear fusion is the powerhouse of the universe.
But it’s long remained elusive for humans to recreate. While nuclear fusion experiments have a 100-year history, most of those have been thought experiments that were far outside of scientists’ reach. In recent decades, however, many governments have started to get serious about cracking the code for nuclear fusion in time to solve our myriad global energy crises and their devastating environmental externalities.
In the South of France, 35 nations have collaborated to make ITER, the world’s largest nuclear fusion reactor, called a tokamak, which involves the use of enormous magnets to heat and control plasma to make a kind of artificial star. ITER has projected that it will achieve first plasma in 2025. China, too, has an enormous state-sponsored tokamak.
And now, for the first time, private companies are also entering the race in earnest. A recent Guardian report identifies this emerging privatization of the nuclear fusion sector to be the single-biggest change in the pursuit of fusion, and perhaps it will be the development that finally tips the scales toward making this once-impossible dream a reality. The Fusion Industry Association, another recent addition to the world of fusion, estimates that private fusion startups have already collectively received more than $2 billion in investments. “Some of the investors in these firms have deep pockets: Jeff Bezos, Peter Thiel, Lockheed Martin, Goldman Sachs, Legal & General, and Chevron have all financed enterprises pursuing this new nuclear power source,” reports the Guardian. “For now, publicly funded labs are producing results a long way ahead of the private firms – but this could change.”
So far, the focus in fusion experiments is still just to show that net energy gain is possible. Until recently, successful fusion experiments required many times more energy than they released. Breakeven energy still hasn’t been achieved, and commercial nuclear fusion will require about 30 times more energy output than energy inputs in order to be viable. Breakeven could easily be 30 years away, but this time that 30-year projection just might stick. And if the global community puts its money and energy behind nuclear fusion to help it advance even more rapidly than its currently impressive pace, commercial fusion may even become a reality in time to play an essential role in avoiding climate change.
By Haley Zaremba for Oilprice.com
A major breakthrough in nuclear fusion has brought us a step closer to ‘infinite’ energy
If development follows this accelerated track, nuclear fusion could amount for about 1% of global energy demand by 2060.
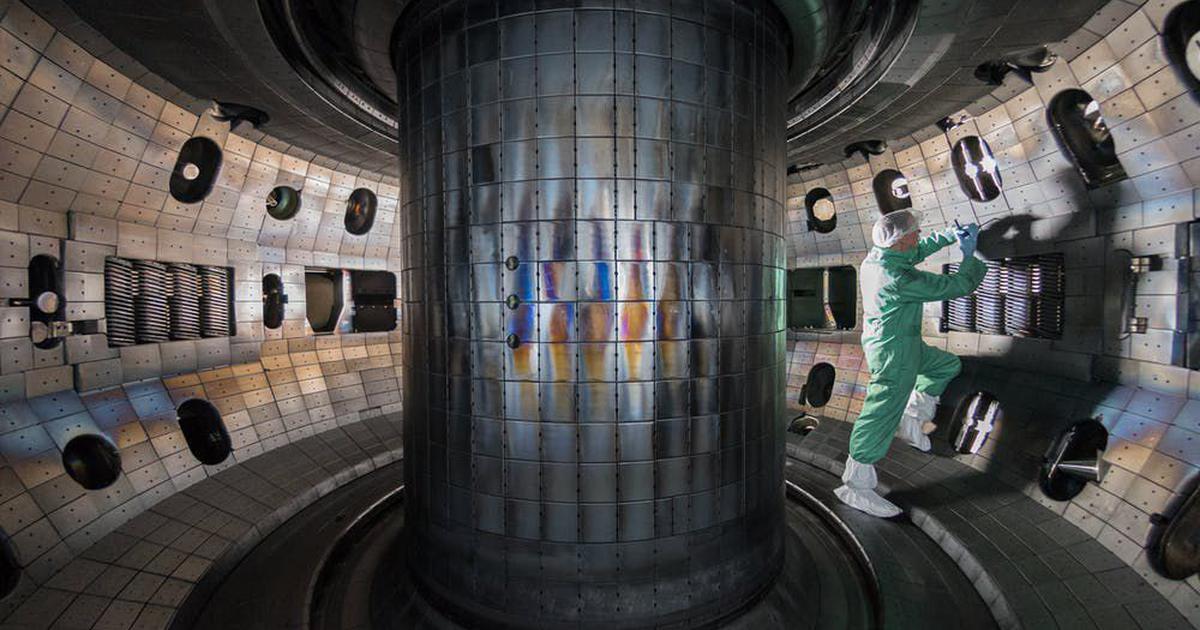
The Lawrence Livermore National Laboratory has announced a major breakthrough in nuclear fusion, using powerful lasers to produce 1.3 megajoules of energy – about 3% of the energy contained in 1kg of crude oil.
Nuclear fusion has long been thought of as the energy of the future – an “infinite” source of power that does not rely on the need to burn carbon. But after decades of research, it has yet to deliver on its exciting promise.
How much closer does this new breakthrough bring us to the desired results? Here is a brief overview to put this new scientific advance into perspective.
What’s nuclear fusion?
There are two ways of using nuclear energy: fission, which is used in current nuclear power plants, and fusion.
In fission, heavy uranium atoms are broken into smaller atoms to release energy. Nuclear fusion is the opposite process: light atoms are transformed into heavier atoms to release energy, the same process that occurs within the plasma core of the Sun.
A fusion reactor amplifies power: the reaction triggered must produce more energy than is needed to heat up the fuel plasma for energy production to occur – this is known as ignition. No one has managed this yet. The current record was achieved in 1997 by the Joint European Torus in the United Kingdom, where 16 megawatts of power were generated by magnetic fusion, but it took 23 megawatts to trigger it.
There are two possible ways of achieving nuclear fusion: magnetic confinement, which uses powerful magnets to confine the plasma for very long periods of time, and inertial confinement, which uses very powerful and brief laser pulses to compress the fuel and start the fusion reaction.
Historically, magnetic fusion has been favoured because the technology needed for inertial fusion, particularly the lasers, was not available. Inertial fusion also requires much higher gains to compensate for the energy consumed by the lasers.
Inertial confinement
The two largest inertial projects are the National Ignition Facility at the Lawrence Livermore National Laboratory in the United States and the Laser MégaJoule in France, whose applications are mainly military and funded by defence programmes. Both facilities simulate nuclear explosions for research purposes, though the National Ignition Facility also carries out research on energy.
The National Ignition Facility uses 192 laser beams that produce a total of 1.9 megajoules of energy for a period lasting a few nanoseconds to trigger the fusion reaction. Fuel is placed inside a metal capsule a few millimetres across, which, when heated by lasers, emits X-rays that heat up and compress the fuel.
It was this process that, on 8 August 2021, achieved the landmark energy production of 1.3 megajoules, the highest value ever recorded by the inertial approach: that is, the closest we have come to ignition.
The overall gain of 0.7 equals the record achieved by Joint European Torus in 1997 using magnetic confinement, but in this case, the fuel absorbed 0.25 megajoules of energy and generated 1.3 megajoules: fusion, therefore, generated a good part of the heat needed for the reaction, approaching the point of ignition.
Still, a reactor will have to achieve much higher gains (more than 100) to be economically attractive.
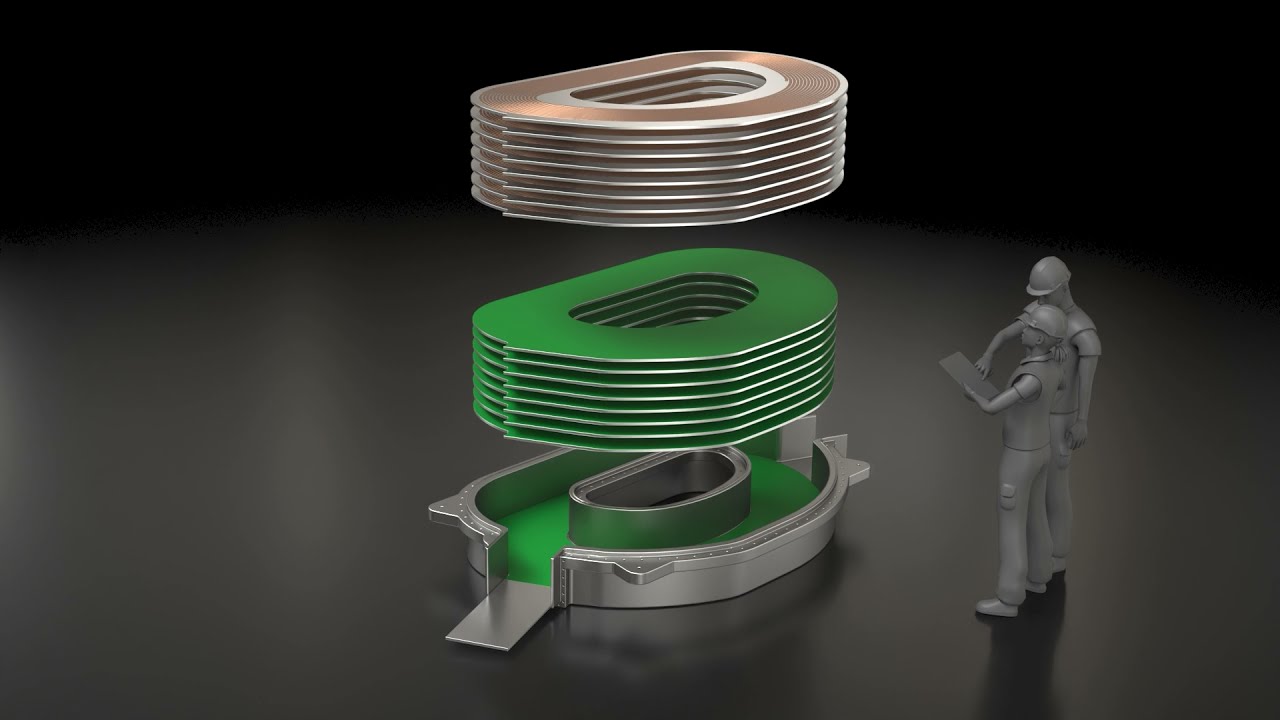
Magnetic confinement
The magnetic confinement approach promises better development prospects and is thus the preferred route for energy production so far.
The vast majority of research focuses on tokamaks, fusion reactors invented in the Soviet Union in the 1960s, where the plasma is confined by a strong magnetic field.
ITER, a demonstration reactor under construction in the south of France involving 35 countries, uses the tokamak configuration. It will be the world’s largest fusion reactor, and aims to demonstrate a gain of 10 – the plasma will be heated by 50 megawatts of power and should generate 500 megawatts. The first plasma is now officially expected by the end of 2025, with a demonstration of fusion expected in the late 2030s.
The UK has recently launched the STEP project (Spherical Tokamak for Electricity Production), which aims to develop a reactor that connects to the energy grid in the 2040s. China is also pursuing an ambitious programme to produce tritium isotopes and electricity in the 2040s. Finally, Europe plans to open another tokamak demonstrator, DEMO, in the 2050s.
Another configuration called the stellarator, like Germany’s Wendelstein-7X, is showing very good results. Though stellarator performances are lower than what a tokamak can achieve, its intrinsic stability and promising recent results make it a serious alternative.
Meanwhile, private nuclear fusion projects have been booming in recent years. Most of t
While these initiatives use other innovative technologies to reach fusion and could thus very well deliver operational reactors fast, deploying a fleet of reactors throughout the world is bound to take time.
If development follows this accelerated track, nuclear fusion could amount for about 1% global energy demand by 2060.
So while this new breakthrough is exciting, it is worth keeping in mind that fusion will be an energy source for the second part of the century – at the earliest.
Greg De Temmerman is an Associate researcher and Managing Director of Zenon Research at Mines ParisTech-PSL.
This article first appeared on The Conversation
No comments:
Post a Comment