By Robert Lea published about 3 hours ago
Three early galaxies discovered by the James Webb Space Telescope could actually be titanic stars powered by a dark matter heart.
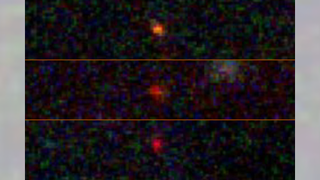
The James Webb Space Telescope (JWST) may have found evidence of a strange and elusive type of star that only existed in the very early universe, when invisible dark matter was one of the only available fuel sources.
New research suggests that three of the earliest objects identified as galaxies by the JWST aren't galaxies at all, but rather "dark stars" — immense, ultrabright hypothetical objects that are powered by dark matter rather than nuclear fusion. If the theory is correct, then this could finally help scientists better understand dark matter, the universe's most mysterious component.
Related: James Webb telescope discovers the 4 oldest galaxies in the universe
"These things are atomic matter that is powered by dark matter, and one supermassive dark star could be as bright as an entire galaxy containing normal fusion-powered stars," astrophysicist Katherine Freese, an astrophysicist at the University of Texas at Austin and lead author of a new study published July 11 in the journal Proceedings of the National Academy of Sciences, told Live Science.

A map of gas and dark matter in a merging galaxy, with blue and green light indicating a gravitationally massive heart of dark matter at the galaxy's center. (Image credit: NASA Goddard)
According to theory, dark stars are enormous in comparison to "ordinary" stars that exist in the universe today, like the sun. Dark stars are hypothesized to have widths hundreds of times greater than the sun's. These stars, composed mostly of hydrogen and some helium, existed in protogalaxies when the universe contained mostly those two elements; heavier elements hadn't yet been forged by nuclear fusion in stars. However, about one thousandth of a dark star’s mass would be made of a secret fuel source — dark matter.
Dark matter, which is all but invisible because it doesn't interact with light, makes up an estimated 85% of the matter in the universe. Theory suggests that when two dark matter particles collide, they may "annihilate" each other, turning their combined mass into a shower of energetic gamma-ray radiation.
"If dark matter is self-annihilating, then the annihilation products could get stuck inside this hydrogen cloud,” that makes up dark stars, Freese said. “And what that means is you're taking all of the energy that used to be in the mass of the dark matter and dumping it into this cloud," Feese said.
Freese added that while "everyday" stars depend on high temperatures, dark matter annihilation could occur at any temperature.
"Dark matter annihilation doesn't care about the temperature," Freese said. "So you have dark matter annihilation throughout the entire [width] of the dark star. And the surface temperature is relatively cool. Because of that, there's no ionizing photons or other stuff coming off preventing the accretion of more matter."
In contrast, when normal stars have acquired enough mass to start nuclear fusion, the radiation that they pump out pushes away the gas envelope that surrounds them, preventing them from accreting more matter and thus growing further.
This means that, while dark stars may start out with a mass about the same as the sun, the objects can accrete more and more matter, growing to be a million times as massive as the sun, and a billion times as bright, Freese added.
Dark star, or ancient galaxy?
Given their huge size, dark stars would appear as more spread-out objects rather than as point-like objects, like modern-day stars. This is how three ancient objects detected by the JWST — namedJADES-GS-z13–0, JADES-GS-z12–0, and JADES-GS-z11–0 — could have been misidentified as galaxies, according to the new research. These candidate dark stars date to between 320 million and to 420 million years after the Big Bang.
But, the dark matter annihilation process can't continue forever. Dark stars sit in the dark-matter-rich centers of protogalaxies, which merge together continuously to form proper galaxies, and eventually, this moves dark stars away from their dark matter fuel.
RELATED STORIES
—James Webb Space Telescope discovers oldest black hole in the universe — a cosmic monster 10 million times heavier than the sun
— 1st image of our galaxy's 'black hole heart' unveiled
—Black holes may be swallowing invisible matter that slows the movement of stars
"As dark stars get displaced from the dark-matter-rich center, the dark stars start collapsing," Freese explained. "This will trigger fusion in the smaller ones, creating ordinary fusion-powered stars [which are all created from collapsing clouds of gas]. The bigger ones will collapse immediately into black holes."
This means that dark stars don't exist in the universe today, Freese added. However, it’s difficult to pinpoint exactly when in the 13.8-billion-year history of the universe that dark stars would have ceased to be.
Confirming the existence of dark stars via these JWST observations would be huge, but Freese pointed out that she and the team aren't quite there yet. This confirmation would require either looking at these candidate dark stars for much longer to build a more complete picture of their light output, or waiting for magnified observations that better reveal the light emissions of these objects, which could allow scientists to identify whether the objects have pure hydrogen and helium compositions, as would be expected from dark stars.
"The dark star idea has been hanging in there for many years, and it would be extremely exciting to me to have this proven correct," Freese concluded.