SPACE/COSMOS
Humans will soon be able to mine on the Moon. But should we? 4 questions to consider
The Conversation
December 31, 2024

Moonlanding
By the end of this decade, nations and private companies may well be mining the surface of the Moon.
But as space becomes accessible to more nations and corporations, we need to stop and ask ourselves what commercial activities we want to allow, including on the Moon.
Now is the time to create the rules and regulations that will protect humanity’s shared future in space and ensure the Moon remains a symbol and inspiration for generations to come.
1. Why mine the Moon?
NASA’s multibillion dollar Artemis program isn’t just about sending astronauts back to the Moon. It’s about paving the way for mining operations.
China is also on a similar trajectory.
All of this has set in motion a new lunar race with private companies competing to figure out how to extract the Moon’s resources, potentially selling it back to governments in a cosmic supply chain.
Currently, all supplies for space exploration are shipped from Earth, making essentials like water and fuel eye-wateringly expensive.
By the time a single litre of water reaches the Moon, its cost beats that of gold.
But by converting water ice on the Moon into hydrogen and oxygen, we can refuel spacecraft on-site. This could make deeper space journeys, especially to Mars, far more feasible.
The Moon’s wealth of rare Earth metals, essential for technologies like smartphones, also means lunar mining could ease the strain on Earth’s dwindling reserves.
Private companies might beat space agencies to the punch; a startup could be mining the Moon before NASA lands its next astronaut.
2. Could mining change how we see the Moon from Earth?
When material is extracted from the Moon, dust gets kicked up. Without an atmosphere to slow it down, this lunar dust can travel vast distances.
That surface material is “space weathered” and duller than the more reflective material beneath. Disturbing the lunar dust means some patches of the Moon may appear brighter where the dust has been kicked up, while other patches may appear more dull if dust resettles on top.
Even small-scale operations might disturb enough dust to create visible changes over time.
Managing lunar dust will be a crucial factor in ensuring sustainable and minimally disruptive mining practices.
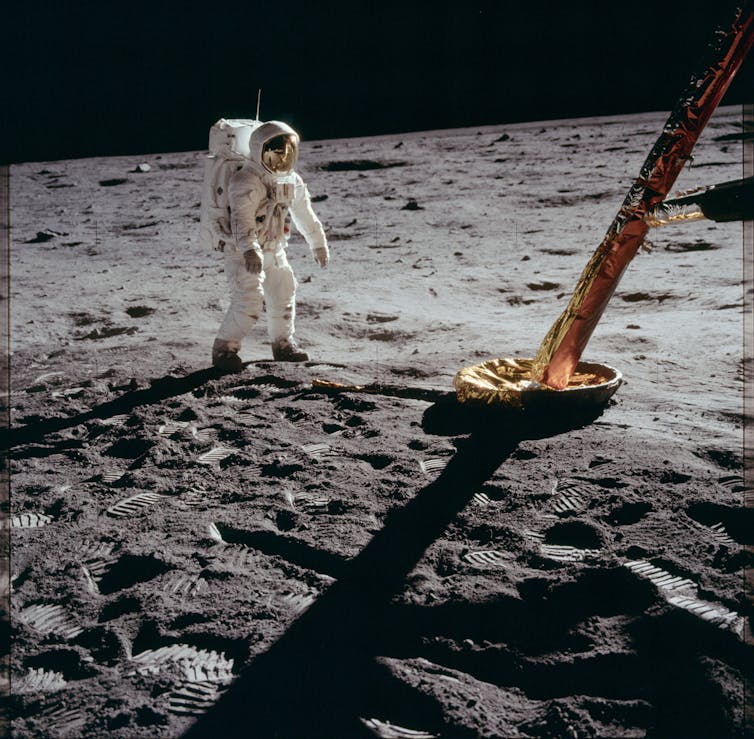
Managing lunar dust will be crucial. Project Apollo Archive/Flickr
3. Who owns the Moon?
The Outer Space Treaty (1967) makes it clear no nation can claim to “own” the Moon (or any celestial body).
However, it is less clear whether a company extracting resources from the Moon violates this non-appropriation clause.
Two later agreements take up this issue.
The 1979 Moon Treaty claims the Moon and its natural resources as “common heritage of mankind”. This is often interpreted as an explicit ban on commercial lunar mining.
The 2020 Artemis Accords, however, allow for mining while reaffirming the Outer Space Treaty’s rejection of any claims of ownership over the Moon itself.
The Outer Space Treaty also notes the exploration of space should benefit everyone on Earth, not just the wealthier nations and corporations able to get there.
When it comes to resource extraction, some argue this means all nations should share in the bounty of any future lunar mining endeavor.
4. What would miners’ lives be like on the Moon?
Imagine you’ve worked 12 hours straight in hot and dirty conditions. You are dehydrated, hungry and overwhelmed. Some of your co-workers have collapsed or been injured due to exhaustion. You all wish you could just get another job with good safety standards, fair pay and reasonable hours. But you can’t. You’re stuck in space.
This dystopian vision highlights the potential dangers of rushing into lunar mining without addressing the risks to workers.
Working in low gravity conditions brings health hazards. Lunar miners are more likely to suffer:
bone and muscle loss
osteoporosis
renal and cardiovascular damage, and
impaired immunity.
Exposure to cosmic radiation not only carries an increased risk of various cancers but can also affect fertility.
Lunar miners will also face prolonged isolation and intense psychological stress. We’ll need good laws and guidelines to protect the health and wellbeing of the space workforce.
Regulatory bodies to enforce worker rights and safety standards will be far away on Earth. Miners may be left with little recourse if asked to work unreasonable hours in unsafe conditions.
British astrobiologist Charles S. Cockell claims this makes space “tyranny-prone”. Powerful individuals could, he argues, be able to abuse people who have nowhere else to go.
The Moon holds incredible promise as a stepping stone for human exploration and a potential source of resources to sustain life on Earth and beyond.
But history has shown us the consequences of unchecked exploitation. Before we mine the Moon, we must establish robust regulations that prioritise fairness, safety and human rights.

Evie Kendal, Senior Lecturer of Health Promotion, Swinburne University of Technology and Alan Duffy, Pro Vice-Chancellor Flagship Initiatives, Swinburne University of Technology
This article is republished from The Conversation under a Creative Commons license. Read the original article.
The carbon in our bodies probably left the galaxy and came back on cosmic ‘conveyer belt’
image:
In this artistic rendering, light from a distant quasar passes through the halo-like circumgalactic medium of a galaxy on its way to Earth, where it is measured by Hubble's Cosmic Origins Spectrograph to determine the composition of the halo.
Credit: NASA/ESA/A. Field
Image URL: https://hubblesite.org/contents/media/images/2011/37/2930-Image.html?news=true
view moreCredit: NASA/ESA/A. Field
Link to full release:
https://www.washington.edu/news/2025/01/03/galaxy-carbon-conveyer-belt/
For immediate release
Friday, Jan. 3, 2025
The carbon in our bodies probably left the galaxy and came back on cosmic ‘conveyer belt’
Life on Earth could not exist without carbon. But carbon itself could not exist without stars. Nearly all elements except hydrogen and helium — including carbon, oxygen and iron — only exist because they were forged in stellar furnaces and later flung into the cosmos when their stars died. In an ultimate act of galactic recycling, planets like ours are formed by incorporating these star-built atoms into their makeup, be it the iron in Earth’s core, the oxygen in its atmosphere or the carbon in the bodies of Earthlings.
A team of scientists based in the U.S. and Canada recently confirmed that carbon and other star-formed atoms don’t just drift idly through space until they are dragooned for new uses. For galaxies like ours, which are still actively forming new stars, these atoms take a circuitous journey. They circle their galaxy of origin on giant currents that extend into intergalactic space. These currents — known as the circumgalactic medium — resemble giant conveyer belts that push material out and draw it back into the galactic interior, where gravity and other forces can assemble these raw materials into planets, moons, asteroids, comets and even new stars.
“Think of the circumgalactic medium as a giant train station: It is constantly pushing material out and pulling it back in,” said team member Samantha Garza, a University of Washington doctoral candidate. “The heavy elements that stars make get pushed out of their host galaxy and into the circumgalactic medium through their explosive supernovae deaths, where they can eventually get pulled back in and continue the cycle of star and planet formation.”
Garza is lead author on a paper describing these findings that was published Dec. 27 in the Astrophysical Journal Letters.
“The implications for galaxy evolution, and for the nature of the reservoir of carbon available to galaxies for forming new stars, are exciting,” said co-author Jessica Werk, UW professor and chair of the Department of Astronomy. “The same carbon in our bodies most likely spent a significant amount of time outside of the galaxy!”
In 2011, a team of scientists for the first time confirmed the long-held theory that star-forming galaxies like ours are surrounded by a circumgalactic medium — and that this large, circulating cloud of material includes hot gases enriched in oxygen. Garza, Werk and their colleagues have discovered that the circumgalactic medium of star-forming galaxies also circulates lower-temperature material like carbon.
“We can now confirm that the circumgalactic medium acts like a giant reservoir for both carbon and oxygen,” said Garza. “And, at least in star-forming galaxies, we suggest that this material then falls back onto the galaxy to continue the recycling process.”
Studying the circumgalactic medium could help scientists understand how this recycling process subsides, which will happen eventually for all galaxies — even ours. One theory is that a slowing or breakdown of the circumgalactic medium’s contribution to the recycling process may explain why a galaxy’s stellar populations decline over long periods of time.
“If you can keep the cycle going — pushing material out and pulling it back in — then theoretically you have enough fuel to keep star formation going,” said Garza.
For this study, the researchers used the Cosmic Origins Spectrograph on the Hubble Space Telescope. The spectrograph measured how light from nine distant quasars — ultra-bright sources of light in the cosmos — is affected by the circumgalactic medium of 11 star-forming galaxies. The Hubble readings indicated that some of the light from the quasars was being absorbed by a specific component in the circumgalactic medium: carbon, and lots of it. In some cases, they detected carbon extending out almost 400,000 light years — or four times the diameter of our own galaxy — into intergalactic space.
Future research is needed to quantify the full extent of the other elements that make up the circumgalactic medium and to further compare how their compositions differ between galaxies that are still making large amounts of stars and galaxies that have largely ceased star formation. Those answers could illuminate not just when galaxies like ours transition into stellar deserts, but why.
Co-authors on the paper are Trystyn Berg, research fellow at the Herzberg Astronomy and Astrophysics Research Centre in British Columbia; Yakov Faerman, a UW postdoctoral researcher in astronomy; Benjamin Oppenheimer, a research fellow at the University of Colorado Boulder; Rongmon Bordoloi, assistant professor of physics at North Carolina State University; and Sara Ellison, professor of physics and astronomy at the University of Victoria. The research was funded by NASA and the National Science Foundation.
###
For more information, contact Garza at samgarza@uw.edu and Werk at jwerk@uw.edu.
An image of a dense, star-rich portion of our galaxy, the Milky Way, taken by the Hubble Space Telescope.
Credit
NASA/ESA/Hubble Heritage Team
Journal
The Astrophysical Journal Letters
Method of Research
Observational study
Subject of Research
Not applicable
Article Title
The CIViL* Survey: The Discovery of a C iv Dichotomy in the Circumgalactic Medium of L* Galaxies
NASA’s LEXI will provide X-ray vision of Earth’s magnetosphere
NASA/Goddard Space Flight Center
image:
In this visualization, the LEXI instrument is shown onboard Firefly Aerospace’s Blue Ghost Mission 1, which will deliver 10 Commercial Lunar Payload Services (CLPS) payloads to the Moon.
view moreCredit: Firefly Aerospace
A NASA X-ray imager is heading to the Moon as part of NASA's Artemis campaign, where it will capture the first global images of the magnetic field that shields Earth from solar radiation.
The Lunar Environment Heliospheric X-ray Imager, or LEXI, instrument is one of 10 payloads aboard the next lunar delivery through NASA’s CLPS (Commercial Lunar Payload Services) initiative, set to launch from the agency's Kennedy Space Center in Florida no earlier than mid-January, with Firefly Aerospace’s Blue Ghost Lander. The instrument will support NASA’s goal to understand how our home planet responds to space weather, the conditions in space driven by the Sun.
Once the dust clears from its lunar landing, LEXI will power on, warm up, and direct its focus back toward Earth. For six days, it will collect images of the X-rays emanating from the edges of our planet’s vast magnetosphere. This comprehensive view could illustrate how this protective boundary responds to space weather and other cosmic forces, as well as how it can open to allow streams of charged solar particles in, creating aurora and potentially damaging infrastructure.
“We’re trying to get this big picture of Earth’s space environment,” said Brian Walsh, a space physicist at Boston University and LEXI’s principal investigator. “A lot of physics can be esoteric or difficult to follow without years of specific training, but this will be science that you can see.”
What LEXI will see is the low-energy X-rays that form when a stream of particles from the Sun, called the solar wind, slams into Earth’s magnetic field. This happens at the edge of the magnetosphere, called the magnetopause. Researchers have recently been able to detect these X-rays in a patchwork of observations from other satellites and instruments. From the vantage point of the Moon, however, the whole magnetopause will be in LEXI’s field of view.
The team back on Earth will be working around the clock to track how the magnetosphere expands, contracts, and changes shape in response to the strength of the solar wind.
“We expect to see the magnetosphere breathing out and breathing in, for the first time,” said Hyunju Connor, an astrophysicist at NASA’s Goddard Space Flight Center in Greenbelt, Maryland, and the NASA lead for LEXI. “When the solar wind is very strong, the magnetosphere will shrink and push backward toward Earth, and then expand when the solar wind weakens.”
The LEXI instrument will also be poised to capture magnetic reconnection, which is when the magnetosphere’s field lines merge with those in the solar wind and release energetic particles that rain down on Earth’s poles. This could help researchers answer lingering questions about these events, including whether they happen at multiple sites simultaneously, whether they occur steadily or in bursts, and more.
These solar particles streaming into Earth’s atmosphere can cause brilliant auroras, but they can also damage satellites orbiting the planet or interfere with power grids on the ground.
“We want to understand how nature behaves,” Connor said, “and by understanding this we can help protect our infrastructure in space.”
s first trip to space. A team at Goddard, including Walsh, built the instrument (then called STORM) to test technology to detect low-energy X-rays over a wide field of view. In 2012, STORM launched into space on a sounding rocket, collected X-ray images, and then fell back to Earth.
It ended up in a display case at Goddard, where it sat for a decade. When NASA put out a call for CLPS projects that could be done quickly and with a limited budget, Walsh thought of the instrument and the potential for what it could see from the lunar surface.
“We’d break the glass — not literally — but remove it, restore it, and refurbish it, and that would allow us to look back and get this global picture that we’ve never had before,” he said. Some old optics and other components were replaced, but the instrument was overall in good shape and is now ready to fly again. “There’s a lot of really rich science we can get from this.”
Under the CLPS model, NASA is investing in commercial delivery services to the Moon to enable industry growth and support long-term lunar exploration. As a primary customer for CLPS deliveries, NASA aims to be one of many customers on future flights. NASA Goddard is a lead science collaborator on LEXI. NASA’s Marshall Space Flight Center in Huntsville, Alabama, manages the development of seven of the 10 CLPS payloads carried on Firefly’s Blue Ghost lunar lander, including LEXI.
Learn more about CLPS and Artemis at:
https://www.nasa.gov/clps
By Kate Ramsayer
NASA’s Goddard Space Flight Center, Greenbelt, Md.
New UVA professor’s research may boost next-generation space rockets
Chen Cui and co-author find hidden ‘shapes’ within EP plasma beams
University of Virginia School of Engineering and Applied Science
image:
Chen Cui is a new associate professor in the Department of Mechanical and Aerospace Engineering at UVA Engineering.
view moreCredit: UVA Engineering
Go faster, farther, more efficiently.
That’s the goal driving spacecraft propulsion engineers like Chen Cui, a new assistant professor at the University of Virginia School of Engineering and Applied Science. Cui is exploring ways to improve electric propulsion thrusters — a key technology for future space missions.
“In order to ensure the technology remains viable for long-term missions, we need to optimize EP integration with spacecraft systems,” Cui said.
Working with his former adviser, University of Southern California professor Joseph Wang, Cui published findings last month in Plasma Sources Science and Technology that provide fresh insights into electron kinetic behavior within plasma beams, perhaps revealing the “shape” of things to come.
The Future of Space Exploration
Cui, who joined the Department of Mechanical and Aerospace Engineering in the fall, focuses his research on understanding how electrons — tiny, fast-moving charged particles — behave in the plasma beams emitted by EP thrusters.
“These particles may be small, but their movement and energy play an important role in determining the macroscopic dynamics of the plume emitted from the electric propulsion thruster,” he said.
By studying these microscopic interactions, Cui aims to better understand how the plume of plasma emitted interacts with the spacecraft itself.
Electric propulsion works by ionizing a neutral gas, usually xenon, and then using electric fields to accelerate the resulting ions. The ions, now forming a high-speed plasma beam, push the spacecraft forward.
Compared to chemical rockets, EP systems are much more fuel-efficient, enabling spacecraft to travel farther while carrying less fuel. These systems are often powered by solar panels or small nuclear reactors, making them ideal for long missions in space, such as NASA's Artemis program, which aims to return humans to the moon, and eventually send astronauts to Mars and beyond.
However, the plume emitted by the thrusters isn’t just exhaust — it’s the lifeline of the entire propulsion system. If not well understood, the plume can cause unexpected problems. Some particles may flow backward toward the spacecraft, potentially damaging important components on the craft, such as solar panels or communication antennas.
“For missions that could last years, EP thrusters must operate smoothly and consistently over long periods of time,” Cui said. This means scientists and engineers must have a deep understanding of how the plasma plume behaves in order to prevent any potential damage.
What the Research Found
Cui specializes in building advanced computer simulations to study how plasma behaves in EP thruster plasma flows. These aren’t just any simulations. They’re powered by modern supercomputers and use a method called Vlasov simulation, an advanced “noise-free” computational method.
The electrons in an EP beam don’t behave exactly as predicted by simple models. They perform differently at different temperatures and speeds, creating distinct patterns.
Being able to precisely see the complexity of electron interactions, while factoring out data that confuse the bigger picture, is key.
“The electrons are a lot like marbles packed into a tube,” Cui said. “Inside the beam, the electrons are hot and move fast. Their temperature doesn’t change much if you go along the beam direction. However, if the ‘marbles’ roll out from the middle of the tube, they start to cool down. This cooling happens more in certain direction, the direction perpendicular the beam’s direction.”
In their most recent paper, they found the electron velocity distribution shows a near-Maxwellian [bell-curve-like] shape in the beam direction and what they describe as a “top-hat” profile in the transverse direction of the beam.
Additionally, Cui and Wang discovered that electron heat flux — the major way thermal energy moves through the EP plasma beam — primarily occurs along the beam’s direction, with unique dynamics that had not been fully captured in previous models.
Publication Information
“Vlasov Simulations of Electric Propulsion Beam,” C. Cui and J. Wang, Plasma Sources Science and Technology, vol. 33, no. 12, p. 125005, 2024.
At UVA Engineering, we are building a brighter future through engineering for the greater good. Our mission is to make the world a better place through bold research and world-class education, putting people at the center of innovation. Our vibrant, diverse community attracts outstanding students and faculty from around the world to our internationally recognized education and research programs, where we are making groundbreaking discoveries in fields including artificial intelligence, hypersonics, cancer research and more. UVA Engineering is part of the top-ranked University of Virginia and is distinguished by a community of care where collegiality, support and engineering ethics thrive.
Journal
Plasma Sources Science and Technology
Method of Research
Computational simulation/modeling
Subject of Research
Not applicable
Article Title
Vlasov Simulations of Electric Propulsion Beam
An Icy Worlds life detection strategy based on Exo-AUV
Peer-Reviewed Publicationimage:
An Icy Worlds Life Detection Strategy
view moreCredit: ©Science China Press
Icy Worlds like Europa and Enceladus provide conditions for the survival of microorganisms. Conducting life detection in regions with high biological potential, such as the icy shell, ice-water interface and seafloor, is likely to discover robust biosignatures, extant life and even prebiotic chemical systems. Extraterrestrial Autonomous Underwater Vehicles (Exo-AUVs) are able to perform in situ, multi-object, multi-scale and multi-dimensional detection autonomously and efficiently. They are expected to serve as crucial tools for planetary scientists and astrobiologists exploring icy worlds and searching for extraterrestrial life.
Based on Europa, it is suggested that the primary science goal of Icy Worlds life detection missions should be the exploration of biological potential which not only aligns with the hypothetical nature of a detection but also helps avoid potential paradoxes associated with binary thinking. By focusing on biological potential, researchers are likely to uncover biosignatures, extant life and even prebiotic chemical systems. The speculation, evaluation and verification of biological potential require consideration of numerous environmental variables and parameters, some of which may serve as biosignatures indicating the presence of life. Just as on Earth, where life thrives in some regions but is scarce in others, detecting the biological potential of Europa should prioritize regions with relatively greater potential for supporting life and biosignatures. Drawing on analogies and ecological theories on Earth, researchers can identify key regions with high biological and biosignature potential, such as the icy shell, ice-water interface and seafloor. However, current detection methodologies often focus on biogenic analysis and overlook strategies for collecting robust biosignatures. In oligotrophic systems, life distribution is sparse and heterogeneous. Even in theoretically promising regions like beneath the ice or on the seafloor, fragile biosignatures may be unable to define biogenesis, regardless by the binary diagnosis or statistical methods.
The process of detecting life on Icy Worlds involves four key procedures: assuming, sampling, analyzing and verifying. The Exo-AUV, along with its ice-penetrating carrier, has the capability to explore the subsurface of the icy shell and carry various payloads for comprehensive data collection and analysis in different dimensions. By applying the ecological niche theory, a life detection strategy for Icy Worlds has been proposed. This strategy guides the Exo-AUV to autonomously identify micro-zones with high biological potential, collect diverse robust biosignatures and potentially detect extant life. The data gathered from Icy Worlds can be used to validate, refute, refine and even reconstruct models based on Earth data. By leveraging the Exo-AUV's underwater detection capabilities, this strategy overcomes limitations of passive data collection and integrates assuming, sampling, analyzing and verifying procedures into a comprehensive methodology for detecting life on Icy Worlds. Ultimately, this strategy aims to uncover robust biosignatures, potential extant life and even prebiotic chemical systems in Europa's thick icy and oceanic layers of hundreds of kilometers thick, with minimal energy and supplies.
Three typical contexts for detecting life on Europa are identified, within the icy shell, at the ice-water interface and on the seafloor. Each context is composed by 4 major contextual elements, environmental conditions, Exo-AUV, the object being measured and key operations. By analyzing these contextual elements along with other pre-procedures such as launching, interplanetary flight, orbit entry and landing, the basic technological requirements for the Exo-AUVs and their ice-penetrating carrier are proposed.
Europa's icy shell and under-ice ocean are both globally distributed. The icy shell thickness may reach about tens of kilometers, with hydrostatic pressure at the deepest ocean floor points potentially doubling that of the Mariana Trench on Earth. Ice-penetrating carriers can utilize Small Modular Reactor (SMR) or Radioisotope Thermal Generator (RTG) power and heat sources, employing a thermal-mechanical hybrid penetrating method and energy-efficient hull design. Navigation assistance can be provided through the use of sonar or synthetic aperture radar, with lateral nozzle jets or auxiliary heat aiding in steering and obstacle avoidance. The carrier penetrates the icy shell to deploy Exo-AUVs into the water, serving as under-ice base station for navigation, communication, data exchange and charging services. The Exo-AUVs are constructed with pressure-resistant hull materials, equipped with RTG power supplies, high-performance navigation and communication modules. They are able to cruise and glide across large space with variable buoyancy, hover around local micro-zones and lean against the undersurface of the icy shell or on the seabed, covering a range from small to large scales.
In order to discover sparse and heterogeneously distributed robust biosignatures and extant life in the vast ice and sea expanse, the Exo-AUV and its ice-penetrating carrier must take a variety of science payloads aboard. These payloads will encompass acoustics, vision, spectroscopy, electrochemistry, analytical chemistry, cell biology and molecular biology instruments. The exploration will gradually focus on objects with different characteristic lengths ranging from several kilometers to sub-micrometers. The collected in situ multi-dimensional information includes morphology, structure, composition, movement, distribution, physiochemistry and etc., and will enables online ecological niche and biogenic analysis. Europa, being far away from Earth, poses challenges due to limited payload capacity of the launch vehicle. The strong radiation from Jupiter above the icy surface demands for protective materials. To address these challenges while ensuring detection capability, microelectromechanical systems (MEMS) technology is employed to achieve payload miniaturing and lightweighting.
The communication delay between Europa and Earth can be as long as 0.5 hours, with a narrow bandwidth and limited window for data exchange. This restricts frequent manual intervention and high-throughput data transfer. In missions focused on detecting complex life, the probe's autonomy becomes crucial. Firstly, Exo-AUV and its ice-penetrating carrier should autonomously localize, navigate and plan the path based on acoustic and optical sensors, and control the propeller, steering rudder and buoyancy to adjust the speed, depth and pose. In addition, based on the detection strategy proposed in this study, Exo-AUV should also achieve science autonomy, speculate potential regions in different scales of space, plan detection tasks, utilize a variety of payloads to complete data acquisition and analysis directly or through onboard tests, verify the assumptions of biological and biosignature potential, update the computational model, summarize, sort and transmit important data independently.
Exo-AUVs developed in the United States and Europe are examined, revealing that current designs lack the capability to tackle intricate life detection tasks and are yet to fully exploit the full potential of the Exo-AUV platform. To prevent stepping into the same old tracks of the Viking missions, a roadmap for conceptual development of Exo-AUVs tailored for detecting life on Icy Worlds is outlined. This roadmap encompasses crucial factors that shape the Exo-AUV concepts. Based on science goals, it is a guideline for the Exo-AUV developers on exploring potential regions, objects detectable and detection strategies, analyzing key contextual elements, refining technological requirements, designing and evaluating concepts with different hull design, payloads and autonomy.
A Concept of Operations for Multiple Exo-AUV System (ConOps for MEAS) is proposed. A simplest MEAS is consisting of an ice-penetrating Exo-AUV Carrier (EAC), a Survey Module-equipped Exo-AUV (EAS) and an Observation Module-equipped Exo-AUV (EAO). The EAC utilizes either an RTG or SMR for power or heat sources and employs a thermal-mechanical hybrid penetrating technique for ice penetration. The EAS and EAO can be housed within the EAC, with all three capable of communication and data sharing through acoustics or fiber optic interfaces. The EAS, featuring a foldable wing-body hull and RTG power supply, is designed for prolonged cruising and gliding within full sea depth, detecting large objects at the ice-water interface and on seafloor. Conversely, the EAO, with a disc-like hull design, full thrusters, rechargeable batteries and various MEMS task payloads, excels at detecting small objects in localized micro-zones. The EAS can connect and disconnect with the EAO in water, acting as a vehicle for transporting, charging and data exchanging. Notably, the MEAS is tailored to address the diverse contextual elements of different potential regions of Europa, where detectable objects and measuring scales vary significantly in size. By distributing technological requirements among the Exo-AUVs, the MEAS efficiently tackles challenges such as idle loads, wasted space, weight, energy and the launch vehicle loading capacity limitations. This concept also enhances maneuverability, robustness, survivability and operational efficiency. In the event of major discoveries, additional MEASs can be launched to create a detection network covering the vast global ice and sea expanse.
A Concept of Operations for Exo-AUV System (ConOps for MESA)
A Roadmap for Conceptual Development of Exo-AUV
Credit
©Science China Press
See the article:
An Icy Worlds life detection strategy based on Exo-AUV, https://doi.org/10.1007/s11430-023-1390-6
Journal
Science China Earth Sciences
No comments:
Post a Comment