Fluid-rich extinct volcanoes cause small earthquakes beneath New Zealand
Imaging of a region where an oceanic tectonic plate descends below another plate reveals evidence that fluid-rich extinct volcanoes can help to lubricate the interface between plates — reducing the potential for large earthquakes.
Catherine A. Rychert &
Nicholas Harmon
At subduction zones, the force of gravity drags dense tectonic plates beneath other, more buoyant plates. As the plates slide past one another, stress builds and is eventually released in the largest and most destructive types of earthquake on Earth. Various factors are thought to have a role in determining the location, type and magnitude of these earthquakes. Working out when and where each of these factors is at play is central to understanding earthquake processes and mitigating the associated hazards. The characteristics of the down-going plate are thought to be a crucial contributor.
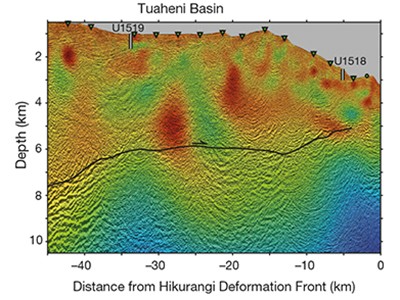
Read the paper: Fluid-rich subducting topography generates anomalous forearc porosity
However, finding direct links between the features of a down-going plate and the associated earthquake characteristics has proved challenging. Chesley et al.1 report in Nature their use of a technique called electromagnetic imaging to investigate extinct underwater volcanoes, known as seamounts, on the Pacific plate as it descends beneath New Zealand. The authors show that the seamounts bring fluid into Earth’s interior that is later released into the overlying plate, effectively lubricating the system and potentially lowering the likelihood of large earthquakes.This use of electromagnetic imaging has produced one of the first high-resolution images of a feature on the down-going slab that directly links the release of fluids to the type and size of earthquakes.
A range of factors dictate the probable location and magnitude of earthquakes2. One such factor is the frictional properties of the interface between the plates. Fluids that are carried into Earth by the down-going plate can reduce the force of friction if they increase the fluid pressure in the fault zone. This, in turn, could decrease the likelihood of large earthquakes3. Alternatively, if the sea floor of the down-going plate is rough, because of features such as seamounts, this might produce areas of higher friction at a fault interface, increasing the likelihood of large earthquakes4.
To investigate this issue, Chesley et al. carried out electromagnetic imaging of the subduction zone in New Zealand. The technology uses either artificial or naturally occurring electric and magnetic fields to determine the degree to which geological features in Earth’s interior are electrically conductive or resistive. The method is particularly sensitive to the presence of interconnected fluids.
The authors produced high-resolution images of a seamount on a section of the Pacific plate that is about to be subducted. They observed that the interior of the seamount is highly conductive, but is overlain by a thin, electrically resistive layer of material (Fig. 1). The authors propose that the conductive interior is fluid-rich, and that the resistive layer is fluid-poor, with low porosity. The resistive layer therefore acts as a cap, limiting the release of fluids from the deeper conductive region until the plate reaches greater depths.
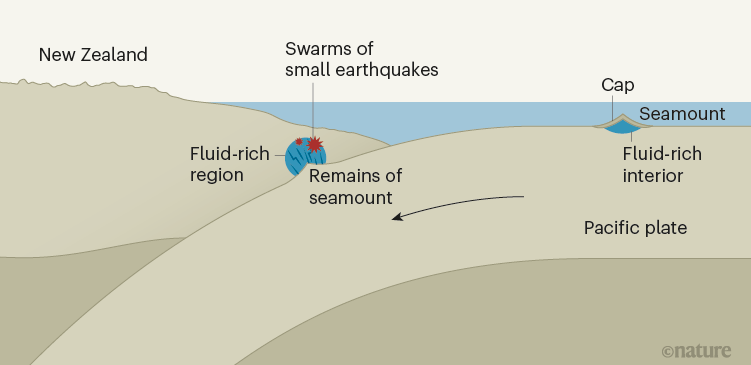
Figure 1 | Evidence that a seamount has altered seismic behaviour beneath New Zealand. The oceanic tectonic plate known as the Pacific plate descends (subducts) beneath the tectonic plate that supports New Zealand. Chesley et al.1 report electromagnetic imaging of a seamount — an extinct underwater volcano — on part of the Pacific plate that is about to subduct, and find that it has a fluid-rich interior capped by a thin, low-porosity layer of material. The authors also observed the remains of a seamount at the interface between the Pacific plate and the overlying plate. The region of the overlying plate above the remains is damaged and fluid-rich, and corresponds to an area in which many small earthquakes have been reported. The authors suggest that the subducted seamount damaged the overlying plate and that its cap broke, releasing fluid to the upper plate. The fluids reduced friction between the plates, thereby decreasing the likelihood of large earthquakes, which often occur at subduction zones.
Chesley et al. also imaged an anomalously resistive feature deeper in the subduction zone, on top of the down-going plate. This corresponds to another seamount that had previously been imaged using seismic waves5,6. The anomaly resides beneath a conductive, fluid-rich region of the over-riding plate and is associated with a swarm of overlying small earthquakes (Fig. 1). The authors conclude that the previously observed seamount was the origin of the fluid in the conductive region now observed over the anomaly, and infer that the small earthquakes occur as the fluid moves through the system. So, although the rough topography of seamounts might be expected to increase friction at faults, seamounts can also decrease friction — and the potential for large ‘mega-thrust’ earthquakes — if they damage the upper plate and release fluids.
Some questions remain. How much fluid is left in the subducted seamount, and how much, if any, is carried deeper into the subduction zone? Deeply transported fluids are also important in subduction-zone settings, because they decrease the melting temperature of the mantle, resulting in hazardous volcanism at the surface7.
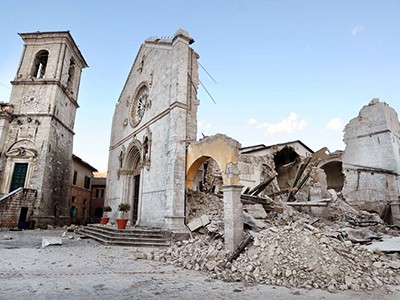
Determining whether the worst earthquake has passed
More work is required to determine the global role of seamounts in influencing subduction dynamics and earthquake hazard. In some locations, seamounts have been associated with aseismic slip8 (movement on faults that does not cause big earthquakes), whereas in other locations they have been linked to large earthquakes4. One explanation for these divergent effects is that seamounts have different hydration levels and are variably fractured around the world; those that are strong, dry and less fractured produce large earthquakes. If this is correct, then what processes cause seamounts to become hydrated or weak? And what is the relative contribution of factors such as sea-floor characteristics, the age of the plate beneath the volcano and the time elapsed since the seamount was an active volcano?
Another unanswered question is, how much fluid do seamounts contribute to subduction zones, compared with other sources of fluid in down-going plates? For instance, processes that occur near sea-floor features called mid-ocean ridges can add fluids to oceanic plates by altering the mineral composition of the rocks9, or through the formation of faults and associated damaged zones10,11, or both. Moreover, further hydration might occur when a plate is bent and broken as it enters the subduction zone12, or, as Chesley et al. suggest, when a local hotspot generates a volcano that eventually becomes a seamount13. The contributions of these diverse mechanisms could vary with location and time. Further imaging of oceanic plates that formed at different times and rates, and with different degrees and times of emergence of volcanic activity, is now required — in particular, by combining electromagnetic and seismic imaging techniques, which have complementary sensitivities to the properties of Earth’s interior.
Nature 595, 178-179 (2021)
doi: https://doi.org/10.1038/d41586-021-01703-7
References
1.
Chesley, C., Naif, S., Key, K. & Bassett, D. Nature 595, 255–260 (2021).
Article Google Scholar
2.
Peng, Z. & Gomberg, J. Nature Geosci. 3, 599–607 (2010).
Article Google Scholar
3.
King Hubbert, M. & Rubey, W. W. Geol. Soc. Am. Bull. 70, 115–205 (1959).
Article Google Scholar
4.
Cloos, M. Geology 20, 601–604 (1992).
Article Google Scholar
5.
Arai, R. et al. J. Geophys. Res. Solid Earth 125, e2020JB020433 (2020).
Article Google Scholar
6.
Bell, R. et al. Geophys. J. Int. 180, 34–48 (2010).
Article Google Scholar
7.
Stern, R. L. Rev. Geophys. 40, 3 (2002).
Article Google Scholar
8.
Bonnet, G., Agard, P., Angiboust, S., Fournier, M. & Omrani, J. Geology 47, 407–410 (2019).
Article Google Scholar
9.
Bach, W. & Früh-Green, G. L. Elements 6, 173–178 (2010).
Article Google Scholar
10.
Cooper, G. F. et al. Nature 582, 525–529 (2020).
PubMed Article Google Scholar
11.
Davy, R. G., Collier, J. S., Henstock, T. J. & the VoiLA Consortium. J. Geophys. Res. Solid Earth 125, e2019JB019100 (2020).
Article Google Scholar
12.
Ranero, C. R., Morgan, J. P., McIntosh, K. & Reichert, C. Nature 425, 367–373 (2003).
PubMed Article Google Scholar
13.
Park, J. & Rye, D. M. Geochem. Geophys. Geosyst. 20, 4779–4809 (2019).
Article Google Scholar
Download references
COMPETING INTERESTS
The authors declare no competing interests.
No comments:
Post a Comment