Part of the Universe’s Missing Matter Found Thanks to Very Large Telescope
- Galaxies exchange matter with their external environment thanks to galactic winds.
- The MUSE instrument from the Very Large Telescope has, for the very first time, mapped the galactic wind that drive these exchanges between galaxies and nebulae.
- This observation led to the detection of some of the Universe’s missing matter.
Galaxies can receive and exchange matter with their external environment thanks to the galactic winds created by stellar explosions. Thanks to the MUSE instrument[1] from the Very Large Telescope at the ESO, an international research team, led on the French side by the CNRS and l’Université Claude Bernard Lyon,[1,2] has mapped a galactic wind for the first time. This unique observation, which is detailed in a study published in MNRAS on September 16, 2021, helped to reveal where some of the Universe’s missing matter is located and to observe the formation of a nebula around a galaxy.
Galaxies are like islands of stars in the Universe, and possess ordinary or baryonic matter, which consists of elements from the periodic table, as well as dark matter,whose composition remains unknown. One of the major problems in understanding the formation of galaxies is that approximately 80% of the baryons[3] that make up the normal matter of galaxies is missing. According to models, they were expelled from galaxies into intergalactic space by the galactic winds created by stellar explosions.
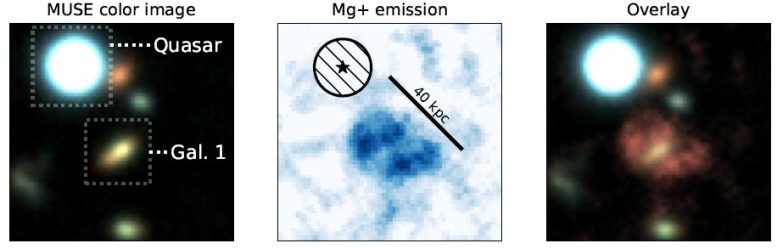
Observation of a part of the Universe thanks to MUSE Left: Demarcation of the quasar and the galaxy studied here, Gal1. Center: Nebula consisting of magnesium represented with a size scale Right: superimposition of the nebula and the Gal1 galaxy. Credit: © Johannes Zabl
An international team,[4] led on the French side by researchers from the CNRS and l’Université Claude Bernard Lyon 1, successfully used the MUSE instrument to generate a detailed map of the galactic wind driving exchanges between a young galaxy in formation and a nebula (a cloud of gas and interstellar dust).
The team chose to observe galaxy Gal1 due to the proximity of a quasar, which served as a “lighthouse” for the scientists by guiding them toward the area of study. They also planned to observe a nebula around this galaxy, although the success of this observation was initially uncertain, as the nebula’s luminosity was unknown.
The perfect positioning of the galaxy and the quasar, as well as the discovery of gas exchange due to galactic winds, made it possible to draw up a unique map. This enabled the first observation of a nebula in formation that is simultaneously emitting and absorbing magnesium—some of the Universe’s missing baryons—with the Gal1 galaxy.
This type of normal matter nebula is known in the near Universe, but their existence for young galaxies in formation had only been supposed.
Scientists thus discovered some of the Universe’s missing baryons, thereby confirming that 80–90% of normal matter is located outside of galaxies, an observation that will help expand models for the evolution of galaxies.
Notes
- MUSE, which stands for Multi Unit Spectroscopic Explorer, is a 3D spectrograph designed to explore the distant Universe. The Centre de recherché astrophysique de Lyon (CNRS/Université Claude Bernard-Lyon 1/ENS de Lyon) led its construction.
- Researchers from the Centre de recherché astrophysique de Lyon (CNRS/Université Claude Bernard Lyon 1/ENS de Lyon), the Galaxies, étoiles, physique, instrumentation laboratory (CNRS/Observatoire de Paris – PSL), and the Institut de recherché en astrophysique et planétologie (CNRS/Université Toulouse III – Paul Sabatier/CNES) participated in the project.
- Baryons are particles consisting of three quarks, such as protons and neutrons. They make up atoms and molecules as well as all visible structures in the observable Universe (stars, galaxies, galaxy clusters, etc.). The “missing” baryons, which had never before been observed, must be distinguished from dark matter, which consists of non-baryonic matter of an unknown nature.
- Including scientists from Saint Mary’s University in Canada, the Institute for Astrophysics at the University of Potsdam in Germany, Leiden University in the Netherlands, the University of Geneva and the Swiss Federal Polytechnic School in Zurich, the Inter-University Centre for Astronomy and Astrophysics in India, and the University of Porto in Portugal.
Reference: “MusE GAs FLOw and Wind (MEGAFLOW) VIII. Discovery of a Mgii emission halo probed by a quasar sightline” by Johannes Zabl, Nicolas F Bouché, Lutz Wisotzki, Joop Schaye, Floriane Leclercq, Thibault Garel, Martin Wendt, Ilane Schroetter, Sowgat Muzahid, Sebastiano Cantalupo, Thierry Contini, Roland Bacon, Jarle Brinchmann and Johan Richard, 28 July 2021, Monthly Notices of the Royal Astronomical Society.
DOI: 10.1093/mnras/stab2165
Telescopes are time machines. Someday, they could take us to a time before starlight.
The Hubble Space Telescope captured some of the oldest light in the universe. Webb will go farther. But what will the telescopes of the future be capable of?
A telescope is like a time machine. When astronomers peer out into the vast distances of space, they’re also looking back in time. That’s because faraway light takes a long time to reach us. When light from distant galaxies enters our telescopes, it’s like a fossil of a time long gone.
Just as scientists study fossils on Earth to understand bygone eras, scientists can chart the evolution of the cosmos by looking at starlight of various ages — but there’s a limit to how far back in time we can see.
The Hubble Space Telescope, which is orbiting the Earth right now, can see 13.3 billion years back in time. Its successor, the James Webb Space Telescope, will be able to peer even farther back in time than the Hubble after it is launched in December
“We are looking for the first light that turned on at the very beginning of cosmic time,” says Caitlin Casey, a UT Austin astronomer who has been approved to use the Webb to look for this light.
This is an epic quest. Humans have never before had the technology to see this first light. It will teach us a lot about how our universe formed, and why it looks the way it does today.
But even this first starlight does not represent the beginning of time. The Big Bang occurred hundreds of millions of years before starlight. For most of that time, the universe was shrouded in darkness. And when there’s no light, there’s nothing for existing telescopes to observe.
Yet astronomers tell me that one day, new and innovative telescopes may be able to see deeper into that darkness — to break through the barriers that even the mighty Webb Space Telescope won’t be able to see through. They dream of putting an observatory in a crater on the far side of the moon, or building one that can potentially detect ripples of warped space coming from the calamity of the Big Bang itself.
When they do, humans may finally be able to piece together a more complete timeline of the history of our universe. And is there any quest more human, or more meaningful, than the drive to understand where we come from?
The space we cannot see
:no_upscale()/cdn.vox-cdn.com/uploads/chorus_asset/file/22884302/9682953582_7029d9580d_b.jpeg)
Let’s start with something very obvious. When we look up at the night sky, we can see the stars because the universe is see-through. Light can travel hundreds of millions of years — billions, even — across the transparent void to reach us. Lucky for us, scientists can use this light to study the history of the cosmos, and our place in it.
But there was a time when our universe was not transparent but opaque. In the beginning, there was darkness.
“When the universe was first created, it was so hot after the Big Bang, atoms couldn’t exist,” explains Paul Hertz, NASA’s director of astrophysics. “It was just a plasma of subatomic particles.”
There’s no light shining through to us from this extreme early universe because light simply cannot travel through a plasma of particles tinier than atoms. Any light that existed then “would just go a very short distance before it would be scattered off some subatomic particle,” Hertz says.
Eventually, several hundred thousand years after the Big Bang, this plasma cooled and a bit of light broke through. Scientists call this light the cosmic microwave background — essentially the afterglow of the Big Bang — and observatories can see it in very dark places like Antarctica, the Atacama Desert, and in Earth’s orbit.
But this light represents only a small snapshot of the early universe, before stars or galaxies. It’s just a nearly uniform dispersal of matter (and dark matter). Somehow, from that starting point, we get everything we see in the universe today.
:no_upscale()/cdn.vox-cdn.com/uploads/chorus_asset/file/22887154/timeline.jpeg)
But soon (cosmically speaking), the universe was dark again. Shortly after the universe cooled, the cosmic dark ages began.
Back in the dark ages, the universe “was full of hydrogen and helium atoms and nothing else,” Hertz says. “And there was nothing to emit light. So it was still dark.”
What’s more, that hydrogen formed “a dense, obscuring fog of primordial gas,” as the National Science Foundation explains. If there was light anywhere, it would be shrouded in the fog.
Only a few hundred million years after the Big Bang was “the darkness of the universe ... pervaded by light for the first time,” Casey says. Astronomers call this period “cosmic dawn,” when the fog lifted and the first starlight shone through the universe. Cosmic dawn, Casey says, permanently transformed the universe “from a dark place to a light place.” That’s the universe we still live in today.
Casey says scientists still don’t precisely know what lifted the fog. They suspect the earliest stars provided the energy needed. That’s what she and others will investigate with the James Webb Space Telescope. “We’re trying to see the first galaxies turn on, for the first time, and emit their light for the first time,” she says.
But the observatory will leave other mysteries untouched: What happened in the dark ages? What happened in the moments after the Big Bang?
This is how, in the future, we might find out.
How special telescopes could see the invisible
How do you see a region of space from which no light emanates? Scientists, surprisingly, have some solutions to this problem.
One is to build a radio telescope on the far side of the moon (that is, the side that never faces the Earth). This type of telescope could help scientists peer into the dark ages, though not necessarily all the way back to the Big Bang.
During the dark ages, astronomers believe, the hydrogen that pervaded the universe emitted very faint radio waves. And that gives astronomers some hope. “You could look back into the dark ages, because those atoms were giving off radio waves,” Hertz says.
It’s as though they were broadcasting a lonesome signal from near the beginning of time, which could make it through the fog.
“If you build the right kind of radio telescope, very large, very sensitive, then you would be able to detect the radio waves and we could study the universe before the first stars and first galaxies,” Hertz says.
:no_upscale()/cdn.vox-cdn.com/uploads/chorus_asset/file/22885255/niac2020_bandyopadhyay.jpeg)
But we can’t detect these faint radio waves from Earth. All the radio transmissions that are produced on Earth would drown them out.
This is where the moon comes in — as a kind of giant shield. The moon is “thousands of miles of rock, so the radio waves can’t get through that,” Hertz explains. The far side of the moon is quiet enough for us to listen in.
Right now there are a few concepts for these moon telescopes, from one that nestles the telescope in a crater to ones that involve lunar rovers. There are currently no concrete plans to build and launch one.
Still, even a giant radio telescope on the far side of the moon could only take us so far. It couldn’t take us all the way back to the Big Bang, when the universe was just a dense plasma of particles.
The world needs more wonder
The Unexplainable newsletter guides you through the most fascinating, unanswered questions in science — and the mind-bending ways scientists are trying to answer them. Sign up today.
Astoundingly, scientists know of something that could get us much closer to the beginning, to make observations of the very early, hot universe as it existed soon after the Big Bang. But it would take a very different type of telescope — one that can see gravitational waves.
The Webb, the Hubble, and even a future radio telescope on the far side of the moon are all telescopes that capture some form of electromagnetism (which include visible light, infrared light, radio waves, microwaves, ultraviolet, and so on).
Gravitational waves, by contrast, are ripples in the very fabric of spacetime. They form as a consequence of the fact that mass can bend space.
And so when massive cataclysms happen in space (say, when two black holes collide), space ripples a bit like after a stone is thrown into a pond. It’s “like flapping space,” Hertz explains. “Space will propagate that movement as waves.”
Gravitational waves are really weird. They literally distort space as they move — shrinking it, stretching it — as if it were an image in a funhouse mirror. The thing is, when these waves reach the Earth, they are almost imperceptibly small, making changes on scales smaller than an individual atom.
/cdn.vox-cdn.com/uploads/chorus_asset/file/6030221/gravitywaves.gif)
Remarkably, scientists have the technology to record gravitational waves. In 2015, scientists first detected gravitational waves that resulted from the collision of two black holes. Scientists believe that there are gravitational waves emanating from this very early, hot universe that existed after the Big Bang, but we would need a huge and specialized observatory to detect them.
“We need to have a gravitational wave observatory where the two ends of it are a million kilometers apart,” Hertz says.
And where do you put such an observatory? In space!
Currently, NASA and the European Space Agency have plans for a space-based gravitational observatory called LISA (the Laser Interferometer Space Antenna), to launch in 2034. It will be a constellation of three satellites that form a triangle, with each side measuring a whopping 2.5 million kilometers.
/cdn.vox-cdn.com/uploads/chorus_asset/file/22885277/slide_09_A1_LISA_orbits2.2021_09_28_14_50_25.gif)
“It measures whether the distance between the satellites has changed,” Hertz explains. “And if it changes, it’s because a gravitational wave went by and shrank or expanded space.”
Some of those gravitational waves could be coming from that hot cauldron of the post-Big Bang universe.
Why build telescopes?
Scientists build telescopes to learn about the history of the cosmos and where we fit into it. When we build observatories to peer into the cosmic dark ages, into the fiery heart of the Big Bang, humans fill in the blank spaces in our timeline of the evolving universe. Telescopes help answer the question: Why does the universe look the way it does today?
This urge to understand may be even deeper and more philosophical than curiosity. As Casey, the UT Austin astronomer, puts it: “Humans trying to understand the universe is really the universe trying to understand itself.”
We humans aren’t separate from the universe. We are of it. The Big Bang, the cosmic dark ages, cosmic dawn ... all of this history led to us.
In this light, building telescopes is a means to make our corner of the universe self-aware.
There’s a virtuous cycle here. The Webb Space Telescope will generate incredible images that are only going to inspire more people to get interested in science, to be curious about the universe they inhabit. Those people may dream up the next barrier-breaking telescope, and the cycle will continue.
There will never be one ultimate telescope that can see everything humans want to see. “Each telescope, whether it’s on Earth or in space, is designed to do a particular kind of science,” Hertz says. Even the mighty LISA, capable of peering back to near the very beginning of time, would be blind to some things, like starlight. Other future telescopes might not set their gaze on the beginning of time, but rather on the many planets that revolve around other stars, as they search for another Earth.
“We’re just a bunch of humans floating on a rock through space,” Casey says. “It’s wild, when you think about it, that we’re able to even piece together what happened before the Earth or the sun even existed.”
Telescopes of the future will bring even more of that history into focus.
The universe should be full of cracks left over from the formation of the universe.
By John Loeffler
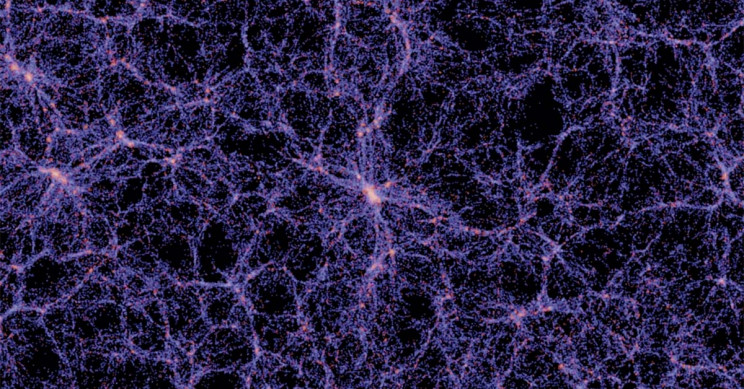
Rich Murray/Flickr
We've all heard about the Big Bang: the explosive emergence of everything in the universe from a single infinitesimally small and infinitely hot point. A point that expanded rapidly and cooled into the galaxies, stars, planets, and eventually people that could look up and observe it all.
It's a hard thing to fathom, but there is evidence of the Big Bang all around us. It comes in the form of cosmic background microwave radiation that permeates the observable universe. And — if some physicists are correct — there are also cracks, like super-thin strings, in the fabric of spacetime caused by the expansion and cooling of the universe.
These cosmic strings, not to be confused with the strings of string theory, would be thinner than a proton but pack immense mass and density, enough to possibly affect the way the early universe developed through their gravitational pull.
Though these strings have likely disappeared over time, it's the effects they may have had on the early universe that scientists hope will reveal their existence and shed light on the conditions of the universe and its evolution in the moments after the Big Bang.
The Universe Right After the Big Bang
About 13.8 billion years ago, the universe could fit into a point smaller than even the smallest subatomic particle, smaller even than the quarks that combine to make up all matter in the universe.
This infinitely tiny point would have been likewise infinitely hot, as all of that matter wasn't matter at all, but a unified superforce: the combined force of gravity, electromagnetic force, weak nuclear force, and the strong nuclear force.
After a Planck unit of time (10-43 seconds, the smallest measurable unit of time possible) from the Big Bang, the temperature of the universe plummetted to a balmy 1029 degrees Kelvin and gravity split off from the other forces.
At around 10-36 seconds, as the initial temperature dropped further, a second fundamental force, the strong nuclear force, separated from the others, leaving the only the electroweak force in a unified state. Cosmic inflation now begins and the universe grows by a factor of 1026, in about the same amount of time as it took after the Big Bang for the strong nuclear force to go its own way.
By around 10-12 seconds after the Big Bang, the temperature cools to around 1012 Kelvin and the last of the fundamental forces, electromagnetic and nuclear weak forces, become distinct. Matter, in the form of quarks and leptons begins to emerge.
For a relatively long time afterward, not much happens, but sometime around 10-6 seconds after the Big Bang, two types of leptons, electrons and neutrinos, are formed.
In the remaining time before the first second had elapsed, the temperature of the universe drops by a factor of 1,000, to a mere 1010 Kelvin, and quarks begin to combine into hadrons. These include the proton and the neutron.
It takes a veritable eternity for helium and other heavier elements to start to form, but they finally do a few minutes after the Big Bang, when the temperature of the universe is about 10,000,000 degrees Kelvin.
Fast forward to about 380,000 years after the Big Bang, and radiation and matter differentiate in a universe that is about 3,000 degrees Kelvin on average.
If you've ever watched Alien 3 and been confused by the fate of the antagonistic Xenomorph at the end, you might not see the problem with this timeline, but superheated objects exhibit strange properties...as anyone who's ever used a ceramic plate to cover a pot of boiling rice (don't ask, it was college and I was dumb) can tell you.
In short, there are things happening to the fabric of spacetime right now because of the extreme temperature.
What are Cosmic Strings?
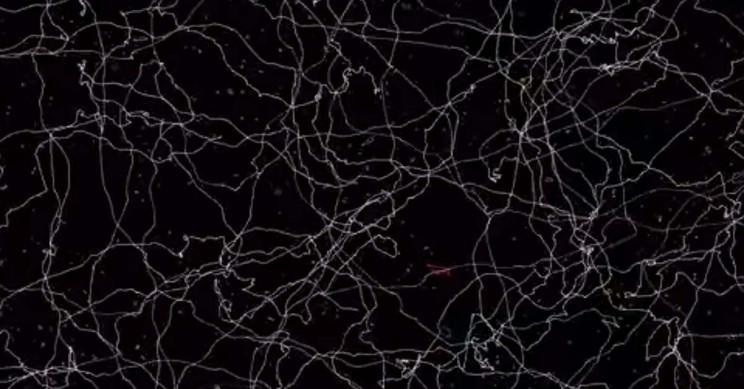
The thing about superheated objects, even the universe, is that they do not cool down evenly.
For context, that ceramic plate mentioned above was about 255 degrees Fahrenheit to the touch at one point on its surface, and it was quickly cooling to room temperature at another point along its edge.
That temperature differential turned my ceramic plate into a bit of a bomb (no one was hurt, thankfully), and the same dynamic was having an interesting effect on the early universe, at least in theory.
As bubbles of cooler universe started to form, their boundaries were coming into contact with other bubbles with different temperatures, creating uneven fault lines between them, akin to cracks in the surface of a frozen lake.
These cracks would have been incredibly thin and of varying lengths, but their mass, and thus their density and gravitational pull, would have been enormous. A mile-long stretch of such a string would have weighed more than the Earth.
These strings would have spiderwebbed their way through the early universe in the first nanoseconds of the universe's existence and become stretched across its entirety as the universe expanded, and the tension of that stretching would have decayed the strings out of existence through gravitational vibration alone.
Some scientists wondered if the magnetic field of these strings stuck around long enough to leave an impression on the universe that could still be observable today. And, in 2010, a research team from the University of Buffalo, NY, published a paper arguing that they'd found just such an artifact in the orientation of ancient quasars.
Have Scientists Been Able to Observe Them?
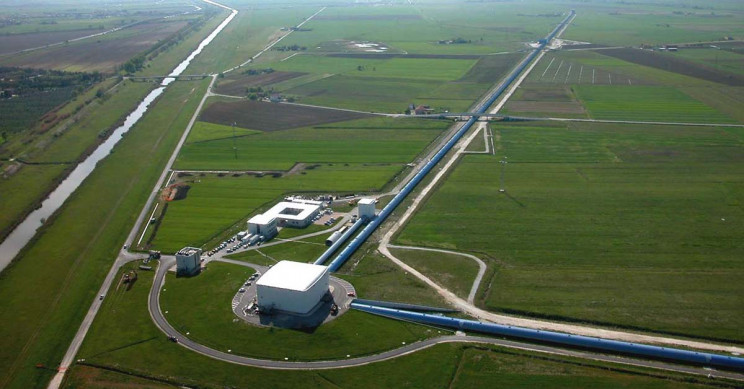
Scientists have not been able to observe these cosmic strings directly, but Robert Poltis and Dejan Stojkovic published a study in the Physical Review Letters that said that a collection of nearly 200 quasars, the supermassive black holes in the center of most galaxies, located in some of the oldest galaxies on record, had their axes oriented in such a way that they formed an arc that was too well-formed to be the result of simply chance.
They suggest that this could have been influenced by the magnetic field of two primordial cosmic strings, which could present some of the first real evidence of their existence.
"It is still early to say that this work has discovered evidence for cosmic strings. It is promising, the science is sound, but one should be careful. There are assumptions made that need be checked," said Jon Urrestilla, of the University of the Basque Country in Biscay, Spain. "But it is yet another piece to the puzzle, and the more predictions we can make from the same basic science into presumably independent effects, the closer we will be to detecting whether strings really were there."
Another promising feature is that since these strings would have formed when the universe was tightly packed into a volume many, many orders of magnitude smaller than its current size, the strings would have been tightly packed together as well in the beginning. But, as the universe expanded, these strings would have stretched out and eventually crossed over one another.
This crossing over would have snapped off parts of these cosmic strings into vibrating, rubber band-like loops whose gravitational ripples might still be measurable in the universe.
A group of researchers at Tufts University ran supercomputer simulations to try to determine how many of these cosmic string loops there might be in the universe and their results, reported in a study published in the journal Physical Review D in 2014, suggested that the number of such cosmic string loops would have been considerable, but fleeting.
According to results from their simulations, there could have been billions of such loops in the universe, raising the possibility that the gravitational waves from these loops could be detected by the Laser Interferometer Gravitational-Wave Observatory (LIGO) or similar gravitational wave detectors.
“The Tufts group has done a heroic job with the string simulations," said Tanmay Vachaspati, an Arizona State University in Tempe physicist who was not involved in the Tufts University study, "and they pin down important features of the loop distribution critical for predicting gravitational-wave emission and their effects on millisecond pulsar timing,”
These gravitational wave signatures have been elusive though, with a major study examing more than 600 days of observation from both LIGO and Virgo gravitational wave observatories failing to record any gravitational waves attributable to a cosmic string loop.
Considering that cosmic strings would be relics of the earliest moments of the universe, researchers have also looked to that other known relic of the early universe, cosmic background radiation, to see if cosmic strings might have left their mark there.
In a 2019 paper published on the preprint server ArXiv [PDF], researchers at McGill University in Montreal found that this is likely not possible with current equipment or techniques, as the marks left by these strings were simply too faint for us to see.
There is still hope that they will turn up eventually, however.
"Many extensions of the Standard Model that people really like — like a lot of superstring theories and others — naturally lead to cosmic strings after inflation [after the Big Bang] takes place," Oscar Hernández, one of the paper's co-authors told Live Science. "So what we have is an object that is predicted by very many models, so if they don't exist then all these models are ruled out. And if they do exist, oh my god, people are happy."
There is hope though that so-called 21-cm imaging will shed more light on cosmic strings, literally. Hydrogen, the most abundant element in the universe and the very first to form shortly after cosmic inflation, radiates electromagnetic radiation at a characteristic 21cm wavelength, and so measuring hydrogen as it gets carried across the universe by cosmic expansion is a promising avenue for identifying cosmic strings.
Measuring that wavelength is still in the early stages since 21cm observatories are just starting to come online, so it's too soon to tell whether this will prove to be the key to identifying these elusive structures somewhere out there in the universe.
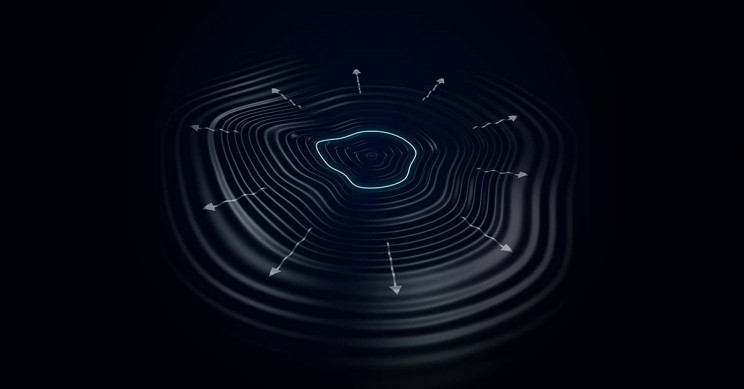
Another exciting bit of potential evidence for cosmic strings comes courtesy of the North American Nanohertz Observatory for Gravitational Waves (NANOGrav).
This observatory gathered data from 45 different pulsars, which shoot out beams of radiation as they spin around their axes, and physicists detected slight variations in the timing of the "pulses" that they emitted. Originally, it was thought that these variations might have been the result of the mergers of pairs of very large black holes, but others argue that these variations might be the evidence of cosmic strings that has proven so elusive.
"We showed that cosmic strings provide a very good fit to the NANOGrav signal, slightly better than the possible alternative source of supermassive black hole binaries," John Ellis and Marek Lewicki, researchers at King's College London and the University of Warsaw, told Phys.org in 2020. "Moreover, we showed that our hypothesis will be straightforward to test in future gravitational wave observatories such as LISA.
Ellis and Lewicki aren't alone either. CERN's Kai Schmitz argued in a recent study in the journal Physical Review Letters, along with co-authors Simone Blasi and Vedran Brdar from the Max-Planck-Institut für Kernphysik, that the gravitational waves recorded in the pulsar data might be something of a gravitational analog of the cosmic microwave background.
"Thus far, all observed signals were caused by astrophysical events such as the mergers of binary black holes," Schmitz told Phys.org. "These events are called 'transient' and only lead to short-lasting signals in gravitational-wave detectors. The next big step in gravitational-wave astronomy is therefore going to be the detection of a stochastic 'background' of gravitational waves, a signal that is constantly present, reaching us from all directions in space."
There could be other causes for such "background" gravitational waves other than cosmic strings, but Schmitz and his co-authors believe that since cosmic strings would be a by-product of the phase transitions from the extremely high-energy state when electromagnetism, the strong force, and the weak force were all unified, they would have permeated the early universe in its infancy and spread across its entirety as it expanded, giving rise to the "background" gravitational waves.
"In this case, the phase transition giving birth to cosmic strings is unlikely to lead to an observable signal in gravitational waves itself, either because it simply does not produce any appreciable signal or because the signal is located at high, unobservable frequencies," Schmitz says.
"Cosmic strings, however, the remnants of the phase transition, have a chance to produce a large signal in gravitational waves that, if detected, can tell us about the symmetries and forces that governed the universe during the first moments of its existence."
Still, healthy skepticism is warranted, as even Schmitz acknowledges: "At present, it is important to remain cautious, as it is not even clear yet whether NANOGrav has really detected a gravitational-wave background."
What if cosmic strings do exist — or at least did exist at some point?
With so many promising avenues to explore, final confirmation of cosmic strings could be right around the corner — or, they might not have existed at all, or at least left no mark that we would ever be able to detect.
According to the LIGO Scientific Collaboration [PDF], cosmic strings would have left a noticeable mark on the cosmic microwave background, which formed roughly 400,000 years after the Big Bang. The gravitational influence of cosmic strings should have woven itself throughout the entire cosmic microwave background, like a lattice, in a way that would still be visible today, but that is not what we see, as Hernández was disappointed to note in his 2019 paper.
"Space-based experiments like [the Cosmic Background Explorer] and [the Wilkinson Microwave Anisotropy Probe] revealed that cosmic strings do not make a measurable contribution to the [cosmic microwave background], thus ruling out a significant role for cosmic strings."
Essentially, even if cosmic strings did exist, their gravitational influence might not have amounted to anything at all in the grand scheme of things.
If they do exist, however, or at least if they did exist at one time and we were able to identify some remnant or artifact of their existence, it would be a significant signpost in the roadmap of the universe; an astronomical remnant of when electromagnetism, the strong force, and the weak force were all united in a single superforce for the most fleeting of moments after our universe burst into existence.
They could also provide some critical support for string theory, which sparked a great deal of excitement after it was first proposed in 1968 but has started to fall out of favor for lack of any solid empirical evidence of its validity.
While cosmic strings are theorized to be incredibly thin structures, they would also be incredibly long, many millions of light-years long, and even possibly extending across the entire observable universe. The strings of string theory, meanwhile, are proposed to be one-dimensional objects on the scale of bosons and fermions, the most fundamental components of matter.
Some hypothesize, though, that a string could exist at a macroscopic scale, so-called F-strings, and exert the same kind of influence on the universe that cosmic strings are theorized to have. If cosmic strings exist, then it would at least give string theorists a solid place to start looking for evidence to bolster a theory that has proven to be almost impossible to test through experimentation.
So, regardless of which side of the string theory divide a physicist is on, finding cosmic strings would be a massive development, making it a strangely unifying endeavor in an often fractious field. The challenge, of course, is getting there.
"If we discover cosmic strings, it’ll be the result of the century,” Eugene Lim, a researcher at King's College London who specializes in the cosmology of the early universe, told Quanta Magazine in 2020. “But to quote Carl Sagan, ‘extraordinary claims require extraordinary evidence,’ and right now the evidence is a bit thin."
No comments:
Post a Comment