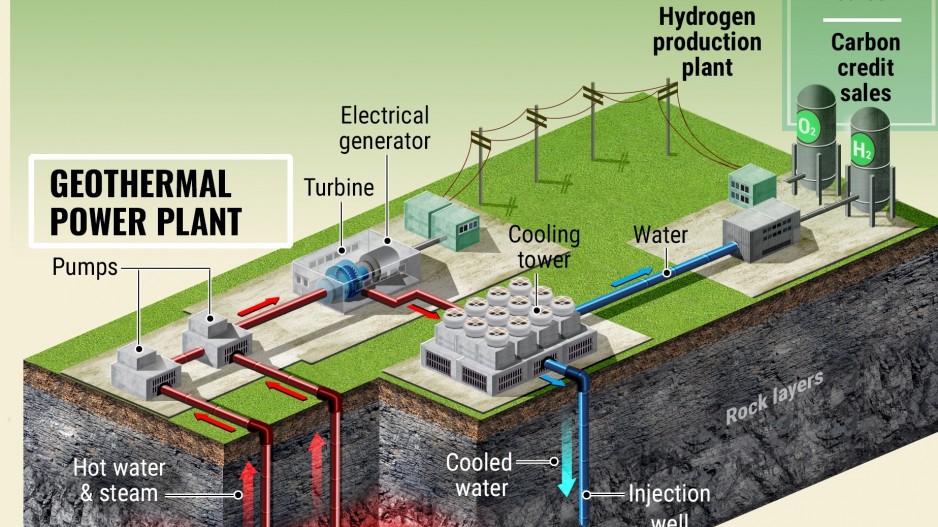
Artist rendering of proposed Meager Creek project
(source: infographic by Meager Creek/ biv.com)
Alexander Richter16 Dec 2021
In an opinion article, Taylor Mattie provided some interesting food for thought as it relates to the challenges to geothermal. His article shared on LinkedIn is here republished with his permission.
For all the of the many reasons, possibilities and opportunities that have led me to dedicate my career to geothermal energy, as a pragmatist, there is also a clear recognition of the primary challenges to holding the concept back from a rapid global embrace: geographic limitations and development cost.
While it is certainly true that technology is advancing rapidly that targets one, or both, of these barriers by enabling deeper drilling, lower temperature resource utilization, closed loops and enhanced geothermal systems, there is one critical and unchangeable fact: geothermal energy is not a commodity.
One cannot ship a barrel of hot water around the world for consumption, as we do with fossil fuels. Of course, geothermal power can be generated and transmitted over long distances, or in some rare cases, such as Iceland, the hot water piped directly over long distances for heating. But in both cases, there are losses that still restrict the economic utilization of a geothermal resource to being a regional source of renewable energy.
Enter green hydrogen.
In recent times, governments and industries alike have recognized the incredible potential that hydrogen can play in global decarbonization, especially in the context of transportation fuel. In this vision, green hydrogen is the apex, as it is defined as hydrogen produced using renewable energy through electrolysis. Most think that solar, wind, and hydro are the renewable power source behind green hydrogen. However, the real golden ticket is baseload geothermal power.
The advantage of geothermal power generation is that it runs full time and is not impacted by whether or not the sun is shining or wind is blowing; it provides consistent, baseload power output capability that makes it a perfect energy provider for green hydrogen facilities that operate in the same manner.
Geothermal and green hydrogen designated as a system is a symbiotic relationship with incredible advantages that only come when they are paired together; baseload power supply maintains hydrogen production 24 hours a day, surface footprint is minimized, and government subsidies can be stacked to enable development. Since green hydrogen can be transported or stored for later use, it is the method that enables the pseudo-commoditization of geothermal energy.
There are many remote locations around the world, and especially in the Pacific Rim “Ring of Fire,” where vast geothermal resources exist but with small local populations, and therefore limited need to utilize these resources to its full potential or have a means to fund the development. However, coupling this with green hydrogen, there is now a new model emerging that greatly increases the attractiveness of geothermal in remote areas.
This is not going unnoticed by the industry as well: the recent Halcyon Project in New Zealand has just been finished, which is a green hydrogen plant powered by local geothermal power. Other projects like Meager Creek Development Corporation in British Columbia are in planning, but there are also some extremely visionary organizations like Fortescue Future Industries who are laser-focused on bringing green hydrogen to the world and relying on massive amounts of geothermal energy to enable it.
Now that the possibilities of enabling a pseudo-commoditization of geothermal with green hydrogen are taking shape, how is the other primary challenge of development cost covered with this partnership?
While there is government policy being implemented in many countries financing and supporting the development of green hydrogen, I’ll focus just on the USA as an example. The new Infrastructure and Jobs Act (IIJA) created an Office of Clean Energy Demonstrations, with a budget of about $21.5 billion dollars, designated to meet a set of 10 goals. While geothermal energy is not mentioned specifically, it can easily be interpreted that several areas of the funding could go to geothermal developments to help meet the greater goals.
Of that $21.5 billion budget, the largest portion, $8 billion, is designed for “Regional clean hydrogen hubs,” which is inclusive of the producers, consumers and infrastructure needed to build out the network. The Office has the goal of developing at least four hubs in different regions of the U.S., with at least one being a green hydrogen facility. (The others are blue hydrogen, fuelled by splitting natural gas into hydrogen and CO2.)
Of course, there are massive power requirements for green hydrogen generation facilities and networks of this size, and it is interpreted that this funding would be inclusive of the power source required. The actual footprint of how a network would look is beyond my area of expertise, but it seems reasonable to think that there would be a mix of centralized/direct power sources; Centralized geothermal power plants for hydrogen generation and compression facilities, and then decentralized facilities (such as H2 fuelling stations) that would purchase the renewable energy from the grid, or simply be powered by fuelled generators on-site.
Many questions still exist. For example, if a geothermal power plant is built 50 miles from a green hydrogen generation facility, is it considered a part of the hydrogen hub and therefore qualify for funding? However, it is expected the criteria and structure is something that will become clearer as we move though 2022. It seems highly plausible that portions of this $8 billion will become available to geothermal developers and lease holders such as Fervo Energy, Cyrq and Ormat. They have a huge potential to benefit from this, as does the industry as a whole.
Geothermal has always had to struggle to gain visibility for the funding needed to expand development. But with an incredible amount of funding going into green hydrogen, “piggybacking” into this area opens doors for a high volume of geothermal development in more geographically diverse regions.
The green hydrogen industry and geothermal industry have an enormous degree of synergy to offer each other and are better together. A goal of unification, both in terms of joint project development and global policy creation, should be established by leaders of both industries to take full advantage of the opportunities at hand and better foster the energy transition.
The thoughts and opinions in this essay are mine and not those of my employer (Baker Hughes).
Source: Taylor Mattie via LinkedIn
Alexander Richter16 Dec 2021
In an opinion article, Taylor Mattie provided some interesting food for thought as it relates to the challenges to geothermal. His article shared on LinkedIn is here republished with his permission.
For all the of the many reasons, possibilities and opportunities that have led me to dedicate my career to geothermal energy, as a pragmatist, there is also a clear recognition of the primary challenges to holding the concept back from a rapid global embrace: geographic limitations and development cost.
While it is certainly true that technology is advancing rapidly that targets one, or both, of these barriers by enabling deeper drilling, lower temperature resource utilization, closed loops and enhanced geothermal systems, there is one critical and unchangeable fact: geothermal energy is not a commodity.
One cannot ship a barrel of hot water around the world for consumption, as we do with fossil fuels. Of course, geothermal power can be generated and transmitted over long distances, or in some rare cases, such as Iceland, the hot water piped directly over long distances for heating. But in both cases, there are losses that still restrict the economic utilization of a geothermal resource to being a regional source of renewable energy.
Enter green hydrogen.
In recent times, governments and industries alike have recognized the incredible potential that hydrogen can play in global decarbonization, especially in the context of transportation fuel. In this vision, green hydrogen is the apex, as it is defined as hydrogen produced using renewable energy through electrolysis. Most think that solar, wind, and hydro are the renewable power source behind green hydrogen. However, the real golden ticket is baseload geothermal power.
The advantage of geothermal power generation is that it runs full time and is not impacted by whether or not the sun is shining or wind is blowing; it provides consistent, baseload power output capability that makes it a perfect energy provider for green hydrogen facilities that operate in the same manner.
Geothermal and green hydrogen designated as a system is a symbiotic relationship with incredible advantages that only come when they are paired together; baseload power supply maintains hydrogen production 24 hours a day, surface footprint is minimized, and government subsidies can be stacked to enable development. Since green hydrogen can be transported or stored for later use, it is the method that enables the pseudo-commoditization of geothermal energy.
There are many remote locations around the world, and especially in the Pacific Rim “Ring of Fire,” where vast geothermal resources exist but with small local populations, and therefore limited need to utilize these resources to its full potential or have a means to fund the development. However, coupling this with green hydrogen, there is now a new model emerging that greatly increases the attractiveness of geothermal in remote areas.
This is not going unnoticed by the industry as well: the recent Halcyon Project in New Zealand has just been finished, which is a green hydrogen plant powered by local geothermal power. Other projects like Meager Creek Development Corporation in British Columbia are in planning, but there are also some extremely visionary organizations like Fortescue Future Industries who are laser-focused on bringing green hydrogen to the world and relying on massive amounts of geothermal energy to enable it.
Now that the possibilities of enabling a pseudo-commoditization of geothermal with green hydrogen are taking shape, how is the other primary challenge of development cost covered with this partnership?
While there is government policy being implemented in many countries financing and supporting the development of green hydrogen, I’ll focus just on the USA as an example. The new Infrastructure and Jobs Act (IIJA) created an Office of Clean Energy Demonstrations, with a budget of about $21.5 billion dollars, designated to meet a set of 10 goals. While geothermal energy is not mentioned specifically, it can easily be interpreted that several areas of the funding could go to geothermal developments to help meet the greater goals.
Of that $21.5 billion budget, the largest portion, $8 billion, is designed for “Regional clean hydrogen hubs,” which is inclusive of the producers, consumers and infrastructure needed to build out the network. The Office has the goal of developing at least four hubs in different regions of the U.S., with at least one being a green hydrogen facility. (The others are blue hydrogen, fuelled by splitting natural gas into hydrogen and CO2.)
Of course, there are massive power requirements for green hydrogen generation facilities and networks of this size, and it is interpreted that this funding would be inclusive of the power source required. The actual footprint of how a network would look is beyond my area of expertise, but it seems reasonable to think that there would be a mix of centralized/direct power sources; Centralized geothermal power plants for hydrogen generation and compression facilities, and then decentralized facilities (such as H2 fuelling stations) that would purchase the renewable energy from the grid, or simply be powered by fuelled generators on-site.
Many questions still exist. For example, if a geothermal power plant is built 50 miles from a green hydrogen generation facility, is it considered a part of the hydrogen hub and therefore qualify for funding? However, it is expected the criteria and structure is something that will become clearer as we move though 2022. It seems highly plausible that portions of this $8 billion will become available to geothermal developers and lease holders such as Fervo Energy, Cyrq and Ormat. They have a huge potential to benefit from this, as does the industry as a whole.
Geothermal has always had to struggle to gain visibility for the funding needed to expand development. But with an incredible amount of funding going into green hydrogen, “piggybacking” into this area opens doors for a high volume of geothermal development in more geographically diverse regions.
The green hydrogen industry and geothermal industry have an enormous degree of synergy to offer each other and are better together. A goal of unification, both in terms of joint project development and global policy creation, should be established by leaders of both industries to take full advantage of the opportunities at hand and better foster the energy transition.
The thoughts and opinions in this essay are mine and not those of my employer (Baker Hughes).
Source: Taylor Mattie via LinkedIn
Researchers pioneer viewing deep rock
fractures for geothermal
Model heat conductivity map (source: PNNL)
Alexander Richter18 Dec 2021
Researchers of the Pacific Northwest National Lab in the U.S. have pioneered a new subsurface imaging technique that allows monitoring deep rock subsurface fractures for geothermal.
Scorching hot granite deep underground can be tapped for energy by opening up cracks in the rock. This potential resource, known as enhanced geothermal energy, requires a clear sense of changes happening in the rock over time—a complex picture that can be difficult to capture, so a news update by the Pacific Northwest National Laboratory (PNNL).
A team led by researchers at PNNL has demonstrated a new way to monitor deep subsurface fractures. The technique, electrical resistivity tomography (ERT), gauges underground changes by measuring electrical conductivity in the rock. ERT produces 4D—that is, 3D plus time-lapse—images of the subsurface.
What is an enhanced geothermal system?
Conventional geothermal systems rely on water and flow pathways that are already present within hot rock. An enhanced geothermal system harvests heat trapped within dry rock by introducing water and cracks. Operators drill two underground wells thousands of feet below the surface and then inject fluid at high pressure to fracture the rock between the wells. The fracturing process for heat is similar to what’s known as “fracking” shale rock to release oil and gas.
Temperatures at this level can reach beyond 200 ºC (392 ºF). Water pumped from one well to the other and back up to the surface collects heat from the rock, generating steam that can drive a turbine for electricity.
Enhanced geothermal systems could provide an estimated 100 gigawatts of electricity—enough to power 100 million homes. But such systems involve expensive drilling, and they need better monitoring and prediction of underground changes to reduce the uncertainty and risk associated with a given project.
Like any underground environment, enhanced geothermal systems change over time. Fractures in the rock open and close in response to stresses caused by high-pressure fluid injections, changing the system’s heat output. Seismic activity is one indicator of subsurface stress, but information from microseismic monitoring is limited.
“In these deep, hot rocks, it’s too expensive to drill enough monitoring wells to understand what’s going on using direct sampling,” said Tim Johnson, a computational scientist at PNNL who co-authored the study. “The primary focus of this project is to better understand, and ultimately to predict, how fractures are going to behave in a high-stress environment when you try to connect them between two wells.”
Getting a clearer underground picture
ERT involves placing metal electrodes within monitoring boreholes, then imaging the conductivity of the rock when electric current is sent between them. Increases in conductivity over time show where fractures are opening; when fractures are narrower or closed, conductivity goes down. Johnson developed software called E4D that operates on supercomputing systems and converts all of this electrical information to an image that looks a bit like a heat map, showing variations in conductivity over time. E4D won an R&D 100 Award in 2016.
Check out an animation of the model created here.
“It’s similar to medical imaging, except that you’re doing a time lapse,” Johnson said. “So you’re watching how things change, and usually the change relates to how the fluid is flowing in the subsurface.”
Johnson and other researchers at PNNL have pioneered the use of ERT as a 3D monitoring tool, and E4D at shallower depths of up to 350 feet, where it has been used to detect and trace contaminants, for example. To test it in the deep subsurface, the team deployed it at the Sanford Underground Research Facility in Lead, South Dakota. The work, which is supported by the Department of Energy (DOE)’s Office of Energy Efficiency and Renewable Energy through its Geothermal Technologies Office, is part of a larger collaborative effort across DOE to enhance access to natural resources and storage in the subsurface. Lawrence Berkeley National Laboratory leads the effort, known as the Enhanced Geothermal Systems (EGS) Collab. Partner labs include PNNL, Sandia National Laboratories, Lawrence Livermore National Laboratory, Idaho National Laboratory, and Los Alamos National Laboratory.
Pioneering a new subsurface imaging technique
The intent of the ERT monitoring at Sanford was to monitor fluid flow, as had been done at shallower levels. But the results initially didn’t seem to align with those earlier uses.
“What we were seeing with the changes in conductivity didn’t make sense in terms of fluid flow,” Johnson said. But if the conductivity wasn’t reflecting the movement of fluids, what was it showing?
After years of hunting for an answer, Johnson found it in scientific papers from the 1960s and 1970s. Researchers at the Massachusetts Institute of Technology and also at Lawrence Berkeley National Laboratory had observed changes in the conductivity of crystalline rocks in response to stress—squeezing the rock in lab experiments made it less conductive. This meant the ERT wasn’t simply following fluid underground. It was charting the opening and closing of fractures in response to stress.
“Once we made that link, everything made sense in terms of what the time-lapse images were doing,” Johnson said.
ERT offers several advantages. With no moving parts and electrodes installed outside the well casing, the equipment is low maintenance and can operate while injections are happening. And the imaging happens in real time, giving facility operators feedback they can use almost immediately, if needed. However, ERT cannot be used with metal wellbore casings, which are ubiquitous in deep subsurface projects.
There are ways around this hurdle, such as using fiberglass casing, coating the casing with a non-metallic epoxy, or using a different, nonmetallic material altogether. But for now, Johnson and team are continuing to improve and test the use of ERT at the Sanford facility.
The paper, “4D Proxy Imaging of Fracture Dilation and Stress Shadowing Using Electrical Resistivity Tomography During High Pressure Injections into a Crystalline Rock Formation,” was published in October in the Journal of Geophysical Research: Solid Earth. Co-authors with Johnson were Jeff Burghardt, Chris Strickland, Hunter Knox, Vince Vermeul, and Mark White at PNNL; Paul Schwering and Doug Blankenship at Sandia National Laboratories; Tim Kneafsey at Lawrence Berkeley National Laboratory; and the team at EGS Collab.
Source: release/ Pacific Northwest National Lab
Alexander Richter18 Dec 2021
Researchers of the Pacific Northwest National Lab in the U.S. have pioneered a new subsurface imaging technique that allows monitoring deep rock subsurface fractures for geothermal.
Scorching hot granite deep underground can be tapped for energy by opening up cracks in the rock. This potential resource, known as enhanced geothermal energy, requires a clear sense of changes happening in the rock over time—a complex picture that can be difficult to capture, so a news update by the Pacific Northwest National Laboratory (PNNL).
A team led by researchers at PNNL has demonstrated a new way to monitor deep subsurface fractures. The technique, electrical resistivity tomography (ERT), gauges underground changes by measuring electrical conductivity in the rock. ERT produces 4D—that is, 3D plus time-lapse—images of the subsurface.
What is an enhanced geothermal system?
Conventional geothermal systems rely on water and flow pathways that are already present within hot rock. An enhanced geothermal system harvests heat trapped within dry rock by introducing water and cracks. Operators drill two underground wells thousands of feet below the surface and then inject fluid at high pressure to fracture the rock between the wells. The fracturing process for heat is similar to what’s known as “fracking” shale rock to release oil and gas.
Temperatures at this level can reach beyond 200 ºC (392 ºF). Water pumped from one well to the other and back up to the surface collects heat from the rock, generating steam that can drive a turbine for electricity.
Enhanced geothermal systems could provide an estimated 100 gigawatts of electricity—enough to power 100 million homes. But such systems involve expensive drilling, and they need better monitoring and prediction of underground changes to reduce the uncertainty and risk associated with a given project.
Like any underground environment, enhanced geothermal systems change over time. Fractures in the rock open and close in response to stresses caused by high-pressure fluid injections, changing the system’s heat output. Seismic activity is one indicator of subsurface stress, but information from microseismic monitoring is limited.
“In these deep, hot rocks, it’s too expensive to drill enough monitoring wells to understand what’s going on using direct sampling,” said Tim Johnson, a computational scientist at PNNL who co-authored the study. “The primary focus of this project is to better understand, and ultimately to predict, how fractures are going to behave in a high-stress environment when you try to connect them between two wells.”
Getting a clearer underground picture
ERT involves placing metal electrodes within monitoring boreholes, then imaging the conductivity of the rock when electric current is sent between them. Increases in conductivity over time show where fractures are opening; when fractures are narrower or closed, conductivity goes down. Johnson developed software called E4D that operates on supercomputing systems and converts all of this electrical information to an image that looks a bit like a heat map, showing variations in conductivity over time. E4D won an R&D 100 Award in 2016.
Check out an animation of the model created here.
“It’s similar to medical imaging, except that you’re doing a time lapse,” Johnson said. “So you’re watching how things change, and usually the change relates to how the fluid is flowing in the subsurface.”
Johnson and other researchers at PNNL have pioneered the use of ERT as a 3D monitoring tool, and E4D at shallower depths of up to 350 feet, where it has been used to detect and trace contaminants, for example. To test it in the deep subsurface, the team deployed it at the Sanford Underground Research Facility in Lead, South Dakota. The work, which is supported by the Department of Energy (DOE)’s Office of Energy Efficiency and Renewable Energy through its Geothermal Technologies Office, is part of a larger collaborative effort across DOE to enhance access to natural resources and storage in the subsurface. Lawrence Berkeley National Laboratory leads the effort, known as the Enhanced Geothermal Systems (EGS) Collab. Partner labs include PNNL, Sandia National Laboratories, Lawrence Livermore National Laboratory, Idaho National Laboratory, and Los Alamos National Laboratory.
Pioneering a new subsurface imaging technique
The intent of the ERT monitoring at Sanford was to monitor fluid flow, as had been done at shallower levels. But the results initially didn’t seem to align with those earlier uses.
“What we were seeing with the changes in conductivity didn’t make sense in terms of fluid flow,” Johnson said. But if the conductivity wasn’t reflecting the movement of fluids, what was it showing?
After years of hunting for an answer, Johnson found it in scientific papers from the 1960s and 1970s. Researchers at the Massachusetts Institute of Technology and also at Lawrence Berkeley National Laboratory had observed changes in the conductivity of crystalline rocks in response to stress—squeezing the rock in lab experiments made it less conductive. This meant the ERT wasn’t simply following fluid underground. It was charting the opening and closing of fractures in response to stress.
“Once we made that link, everything made sense in terms of what the time-lapse images were doing,” Johnson said.
ERT offers several advantages. With no moving parts and electrodes installed outside the well casing, the equipment is low maintenance and can operate while injections are happening. And the imaging happens in real time, giving facility operators feedback they can use almost immediately, if needed. However, ERT cannot be used with metal wellbore casings, which are ubiquitous in deep subsurface projects.
There are ways around this hurdle, such as using fiberglass casing, coating the casing with a non-metallic epoxy, or using a different, nonmetallic material altogether. But for now, Johnson and team are continuing to improve and test the use of ERT at the Sanford facility.
The paper, “4D Proxy Imaging of Fracture Dilation and Stress Shadowing Using Electrical Resistivity Tomography During High Pressure Injections into a Crystalline Rock Formation,” was published in October in the Journal of Geophysical Research: Solid Earth. Co-authors with Johnson were Jeff Burghardt, Chris Strickland, Hunter Knox, Vince Vermeul, and Mark White at PNNL; Paul Schwering and Doug Blankenship at Sandia National Laboratories; Tim Kneafsey at Lawrence Berkeley National Laboratory; and the team at EGS Collab.
Source: release/ Pacific Northwest National Lab
No comments:
Post a Comment